Early screening of cancer is desirable as most tumors are detectable only when they reach a certain size when they contain millions of cells that may already have metastasized. Currently employed diagnostic techniques such as medical imaging, tissue biopsy and bioanalytical assay of body fluids by enzyme linked immunosorbent assay (ELISA) are insufficiently sensitive and specific to detect most types of early-stage cancers. Moreover, these assays are labour intensive, time consuming, expensive and don’t have multiplexing capability. On the other hand, QD based detection is rapid, easy and economical enabling quick point-of-care screening of cancer markers. QDs have got unique properties which make them ideal for detecting tumors. These include intense and stable fluorescence for a longer time; resistance to photobleaching [1-5], large molar extinction coefficients, and highly sensitive detection due to their ability to absorb and emit light very efficiently. Due to their large surface area-to-volume ratio, a single QD can be conjugated to various molecules, thus making QDs appealing for employment in designing more complex multifunctional nanostructures. Various types of biomarkers such as proteins, specific DNA or mRNA sequences and circulating tumor cells have been identified for cancer diagnosis from serum samples. Therefore, QD based multiplexed approach [1] for the simultaneous identification of many biomarkers would lead to more effective diagnosis of cancer. QDs have been covalently linked to various biomolecules such as antibodies, peptides, nucleic acids and other ligands for fluorescence probing applications [6-19]. Some of the applications of QDs in biology [20-32] along with their tremendous potential for in vivo molecular imaging [33-37] have already been explored.
Advantages of Inorganic Quantum Dots over Organic Fluorophores
Compared to traditional organic fluorophores used for fluorescence labelling in biological experiments, inorganic QDs have wider applications due to their high resistance to photobleaching, which enables visualization of the biological material for a longer time. Fluorophores are highly sensitive to their local environment and can undergo photobleaching, an irreversible photooxidation process which makes them non-fluorescent. This is the main limitation for all studies in which the fluorophore labelled structure has to be observed over extended periods of time. Fluorophores can be optically excited only within a narrow range of wavelengths and fluorescent emission is also restricted to a certain range of wavelengths. Whereas QDs can be excited with a single light source having wavelength shorter than the wavelength of fluorescence. The fluorescence spectra of QDs are narrow, symmetric and have no red tail as observed in fluorophores. Various colors can be observed and distinguished without any spectral overlap. Therefore, multicolor labelling of different structures with QDs of different colors became possible. This multiplexed approach [3, 38-40] is of great interest in wide ranging applications such as disease diagnosis and drug delivery.
The field of QDs is of multidisciplinary as persons from different scientific disciplines i.e. chemistry, physics, biology and medicine are working together to harness their potential. Their employment for the detection and treatment of cancer is one such application which is of paramount importance.
Quantum Dot Technology
QDs are inorganic semiconductor nanocrystals having typical diameter between 2-8 nm that possess unique luminescent properties. They are generally composed of atoms from groups II and VI elements (e.g. CdSe and CdTe) or groups III and V elements (e.g. InP and InAs) of the periodic table. Their physical dimensions are smaller than the exciton Bohr radius [1] that leads to quantum confinement effect, which is responsible for their unique optical and electronic properties.
Synthesis of Quantum Dots
High quality QDs have been synthesized by various approaches [41-43]. But usually their synthesis is carried out in organic solvents such as toluene or chloroform at higher temperatures in the presence of surfactants. But the surfactant-coated particles are not soluble in water as they have polar surfactant head group attached to the inorganic core of QD and the hydrophobic chain protruding into the organic solvent. Usually, all experiments with cells involve water-soluble materials. Therefore, various strategies have been developed to make them water-soluble, where either the surfactant layer is replaced or coated with additional layer such as hydrophilic or amphipathic polymers [44-45]. The hydrophobic coating of surfactant is replaced by ligand molecules carrying functional groups at one end that bind to the QD surface, and hydrophilic groups at other end that make the QDs water soluble. The employment of amphiphilic polymers as an additional coating on QD surface has also been reported [38, 46-48]. The hydrophobic tail of the polymer reacts with the hydrophobic surfactant layer on QD surface whereas the hydrophilic groups of the polymer on the outer end impart water solubility. QDs have also been encapsulated in phospholipid micelles [8] to make them water soluble.
Properties and Applications of Quantum Dots
The most commonly used QD system is the inner semiconductor core of CdSe coated with the outer shell of ZnS. The ZnS shell is responsible for the chemical and optical stability of the CdSe core. QDs can be made to emit fluorescent light in the ultraviolet to infrared spectrum just by varying their size. The wavelength of fluorescence of the QD depends on its energy gap (i.e. the difference between the excited and the ground state) which is determined by the size of the QD [49-52]. QDs have narrow spectral line widths, very high levels of brightness, large absorption coefficients across a wide spectral range, high photostability and capability of multiplexed detection. They are very bright and stable even under complex in vivo conditions that make them suitable for advanced molecular and cellular imaging, drug delivery and for highly sensitive bioassays and diagnostics [53-54]. Highly sensitive real-time imaging with greater resolution and tracking of single receptor molecules on the surface of living cells have been made possible by QD bioconjugates [13, 55]. Various applications of quantum dots are stated in figure 1. In most of the cases, functional QD conjugates for cancer detection are composed of a semiconductor core (CdSe, CdTe); an additional shell such as ZnS in the case of CdSe QDs having a higher band gap than CdSe to increase quantum yield; a water soluble hydrophilic coating; and, functionalized antibodies or other biomolecules complementary to the target cancer markers at the tumor sites.
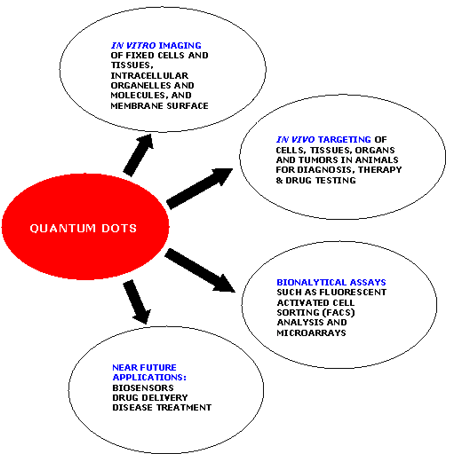
|
Figure 1. Applications of Quantum dots.
|
Overcoming the Toxic Nature of Quantum Dots
The native QDs made up of semiconductor nanoparticles are toxic in nature. It has been observed that CdSe QDs are highly toxic to cells exposed to UV for a longer time [56] as UV dissolves the CdSe, thereby releasing toxic cadmium ions. However, polymer-coated QDs are non-toxic in the absence of UV as demonstrated by in vivo studies [48]. It has also been shown that the micelle-encapsulated QDs injected into the frog embryo did not affect its development [8]. Therefore, QDs are normally encapsulated inside the outer coating of amphiphilic polymers [57-58] to make them water-soluble and resistant to chemical or enzymatic degradation. They are typically synthesized in organic solvents such as tri-n-octyl-phosphine oxide (TOPO) [59-62] and hexadecylamine, having long alkyl chains and high boiling points, to prevent the formation of aggregates. In the recent years, there has been a great development to modify the surface chemistry of QDs to make them water soluble [63-64]. Most commonly, QDs are linked to polyethylene glycol (PEG) or similar ligands to make them biocompatible and to reduce nonspecific binding. They are made specific to the target site by conjugating them to various bioaffinity ligands such as peptides, antibodies, oligonucleotides etc. using different strategies. A possible schematic of the QD bioconjugate for the detection of tumor cell biomarkers is shown in figure 2. Figure 3 describes in brief the various steps of the QD technology for the in vivo diagnosis of cancer.
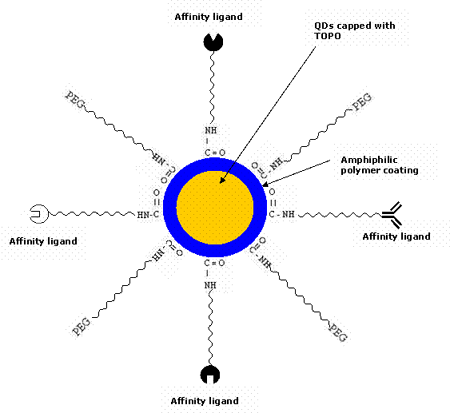
|
Figure 2. Multifunctional QDs usually employed for targeting tumor cells. QDs are conjugated to various affinity ligands (peptide, antibody, inhibitor, drug etc.) specific for the tumor cell biomarkers.
|
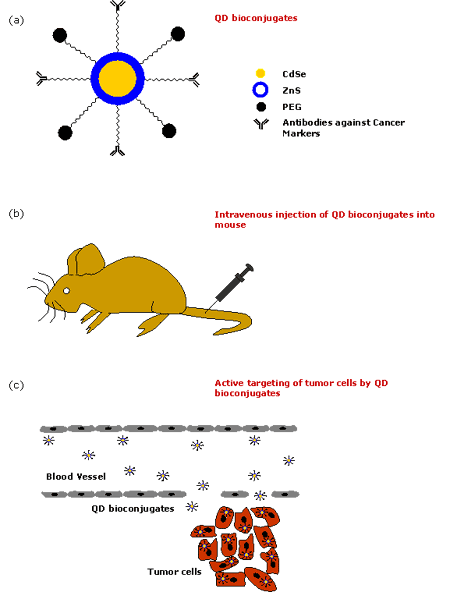
|
Figure 3. Various steps in employing QDs for in vivo diagnosis of cancer. (a) Formation of QD bioconjugates, (b) Intravenous injection of QD bioconjugates into mouse, (c) Active targeting of tumor cells by QD bioconjugates.
|
Blinking Behavior of Quantum Dots
Nirmal et al. [65] discovered for the first time that QDs shows a blinking behavior i.e. intermittent on–off emission upon continuous excitation, which was attributed to Auger ionization [65-66]. The principle of this behavior is not well understood even today. But it is a concern only when a signal from individual QD is required during the analysis such as flow cytometry applications. In such cases, it may be possible that the emission from the individual QD might be off due to ‘blinking’ thus leading to the missing of signal at the detector. But generally in most of the applications such as in cell-based assays, there are more than one QD involved and even if some QDs are blinking, others are giving signal for the final detection and thus, no signal will be missed by the detector. One way of counteracting the reduced quantum yield due to blinking is to grow a shell of a few atomic layers of a material with a larger band gap on top of the QD core.
Effect of Surface Functionalization on the Optical Properties of Quantum Dots
Fundamental studies have revealed that luminescence of QD is very much sensitive to the surface functionalization procedures as the interactions of the molecule with the QD’s surface change the surface charges on the QD [67]. But many of the QD based probing applications are based on the change in fluorescence of QD after the interaction of the target analyte molecules with the biomolecules functionalized on the QD surface. It has been well reported that the surface functionalization of QDs improves their solubility. But it could reduce their quantum efficiency as well. This has demonstrated in the case of meracptoacetic acid-treated QDs where the quantum efficiency was reduced drastically [7, 63]. But protein functionalized quantum dots tend to retain their quantum efficiency and offer longer shelf life. They can also be further functionalized with multiple functional groups [7] without decreasing their quantum efficiency.
Measurement System for Observing and Tracking Quantum dots
Single QDs can be observed and tracked for greater time duration up to a few hours with confocal microscopy, total internal reflection microscopy or epifluorescence microscopy. The scheme of the fluorescent imaging employing QDs as labels and its measurement has been described by Gao et al. [68] and So et al [69]. Gao et al. employed a whole-body macro-illumination system with wavelength-resolved spectral imaging, which allows high sensitivity detection of molecular targets in vivo. So et al. also employed the wavelength-resolved spectral imaging system having software that separated autofluorescence from quantum dot signals.
Active and Passive Quantum Dot Targeting Mechanisms
QD bioconjugates can be delivered to tumors in vivo by both active and passive targeting mechanisms although the passive targeting is much slower and less efficient than active targeting. In the passive targeting mechanism, QD bioconjugates accumulate preferentially at tumor sites due to enhanced permeability and retention effect [70-72]. This effect can be attributed to the facts that angiogenic tumors (i) produce vascular endothelial growth factors, which are responsible for enhanced permeability, (ii) lack an effective lymphatic drainage system, which results in QD bioconjugates accumulation. On the other hand, in the active targeting mechanism, antibody-conjugated QDs are employed where the antibody gets attached to their specific tumor biomarkers such as prostate specific membrane antigen present on the tumor cells at the target site.
Deep Tissue Imaging Requirements
It has been shown that deep tissue imaging requires the use of far-red and near-infrared light [73]. This necessitates the employment of near-infrared-emitting QDs to increase the tumor imaging sensitivity as the major absorption peaks of blood and water [74] would not interfere in this region.
Removal of Quantum Dots from Living Cells
The clearance of QDs from the living animals and their metabolism demands careful attention and in-depth study before the technology can be used in humans for the diagnosis and treatment of cancer. The only way of clearance of protected QDs from the body is by slow filtration and excretion through the kidney as chemical or enzymatic breakdown is highly unlikely.
Potential Applications of Quantum Dots in Disease Diagnosis and Treatment
Near future will see many potential applications of QDs in the field of disease diagnosis and treatment based on the recent advances in the QD technology and the tremendous interest among researchers.
Conjugation of Biomolecules to Quantum Dots
Various covalent and non-covalent strategies (as shown in figure 4) have been developed for conjugating biomolecules such as proteins and antibodies to the QDs. Biomolecules can be bound covalently employing crosslinkers [1, 6, 8, 17, 38, 44, 64, 75-77], which crosslink the functional groups such as –COOH, -NH2 or –SH present on the QD surface to the functional groups present on the biomolecules. Now-a-days, various conjugation chemistries are available for modifying biomolecules to have the required functional groups.
 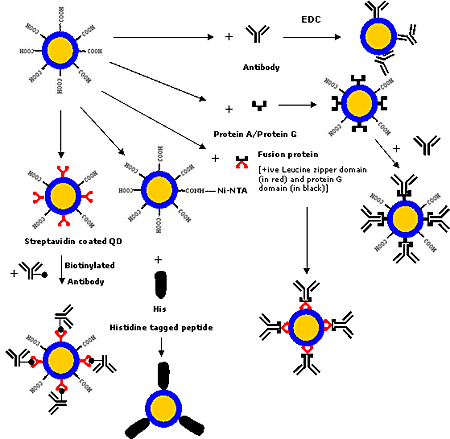
|
Figure 4. Various strategies for conjugating antibodies/proteins to QDs.
|
Strategies to Modify Biomolecules
One strategy employs N-ethyl-N′-(3-diethylaminopropyl) carbodiimide (EDC) as a heterocrosslinker, which crosslinks the carboxylate group of the QDs to the amine group of the proteins. This method does not require any chemical modification of the proteins as most of the proteins contain primary amine.
Another strategy is based on the active ester maleimide-mediated coupling of amine and sulfhydryl groups. But this method has a limitation that the free sulfhydryl groups, which are unstable in the presence of oxygen, are rarely found in native biomolecules. Recently Pellegrino et al. [46] employed preactivated amphiphilic polymer containing multiple anhydride units, which are highly reactive towards primary amines, for binding proteins to QDs. This method has potential applications for making sustained drug delivery system as polyanhydrides are biodegradable polymers. But strategies for the precisely controlled and oriented binding of biomolecules to QDs has have not been much explored. Goldman et al. [78] employed a fusion protein for binding immunoglobulin G (IgG) to QDs. The fusion protein had a positively charged leucine zipper domain that bound electrostatically to the negatively charged QDs and a protein G domain that bound to the constant Fc region of IgG thus leaving the F(ab′)2 region free for antigen binding. A technique based on the targeting of Ni-nitriloacetic acid moieties against hexahistidine motifs, as employed in case of dyes [79], may be employed for binding hexahistidine-tagged biomolecules to QDs using nickel-nitrilotriacetic acid (Ni-NTA) as the chelating agent. Gao and his group at Emory University, USA are working on the development of this technique which would have advantages in terms of controlled oriented binding of biomolecules, compact probe size and low production costs. Streptavidin-biotin binding strategy could also be employed for binding biomolecules to QDs as streptavidin-coated QDs are commercially available and can be readily conjugated to biotinylated biomolecules [13, 38, 55, 59, 80]. Various bioconjugation strategies for QDs are shown in figure 4.
Effects of Quantum Dots on Biological Functions of Biomolecules
It has been shown that in many cases, the conjugation of biomolecules to QDs does not change the binding ability of the biomolecules to their specific receptors [6, 8-9, 13, 17, 38, 55, 58-59 64, 76-77, 80-81] and their biological function. Kloepfer et al [77] observed that the conjugation of QDs to transferrin did not affect the protein function. Dahan et al [82] also observed that the binding of QDs to membrane-bound receptors had no effect on the diffusion behaviour of the receptors in membranes. However, there are few reports that QDs might affect the biological functions of biomolecules such as the binding affinity of neurotransmitter serotonin to serotonin-transporter proteins [14]. This may be due to the steric hindrance of the QDs. Detailed studies are required to investigate the possible effects of QDs on the biological functions of biomolecules.
Advances in Quantum Dot Technology for the Diagnosis of Cancer
In the early stages, QDs were employed for several imaging applications in place of organic dyes. But the tremendous potential of these materials was realized when it was observed that they kept on emitting intense fluorescent light for weeks. This was a major technological advancement for microscopic imaging, which helped in unfolding many cellular processes. In the subsequent stages of development, researchers developed a keen interest in the QD technology and started exploring their applications in different fields. Different QDs composed of the same material but of different sizes had been made, which can generate different colors after activation by light of a single wavelength. It was then demonstrated that QDs tagged with biomolecules such as antibodies, peptides etc. can be employed to detect specific molecules on the cell surface or inside the cell.
Quantum Dot-Peptide Conjugates Shown to Target Tumour Cells
The use of QD-peptide conjugates to target tumor vasculatures in vivo was reported by Akerman and coworkers [58]. They employed ZnS-capped CdSe QDs and showed the targeting capabilities of QDs coated with different peptides. QDs coated with a lung-targeting peptide accumulated in the lungs of the mice after intravenous injection. The peptide got bound to membrane dipeptidase on the endothelial cells in lung blood vessels. In the second case, QDs coated with a targeting peptide got bound to blood vessels and tumor cells in certain tumors. In the third case, QDs coated with a targeting peptide got bound to lymphatic vessels and tumor cells. The group also showed that adding PEG to the outer coating of the QDs prevented nonselective accumulation of QDs in reticuloendothelial tissues.
Quantum Dots Able to identify Live Breast Cancer Cells
A research team from Quantum Dot Corporation and Genentech proved the potential of QDs to identify live breast cancer cells [38] that are likely to respond to an anti-cancer drug. They employed QDs linked to immunoglobulin G (IgG) and streptavidin to label Her2 cancer marker present on the surface of live breast cancer cells and also explored the QD technology for the simultaneous labelling of Her2 on the cell surface and in the nucleus. The researchers simultaneously detected two cellular targets with a single excitation wavelength thereby showing that different colored QDs i.e. QDs of different sizes but same materials could be used together to distinguish different parts of a single cell thus leading to multiplex target detection.
Multifunctional Quantum Dots Simultaneously Target and Image Tumours in Living Animals
Gao and coworkers reported multifunctional QDs for the simultaneous targeting and imaging of tumors in living animals [68]. Highly stable QD conjugate was made up of an amphiphilic triblock copolymer (for in vivo protection), targeting ligands (for tumor antigen recognition), and multiple PEG molecules (for improved biocompatibility and circulation). The in vivo behavior of the QD probes was monitored by tissue section microscopy and whole-animal spectral imaging. QD conjugates were injected intravenously in mice. It was observed that they accumulated at the targeted tumor sites by passive targeting mechanism, due to the leaky nature of tumor blood vessels, and active targeting mechanism, due to the interaction of QD conjugate coated with tumor-specific antibody with the tumor marker. Gao and coworkers also employed QDs to label specific cells in culture and it was observed that within a small period of time, QDs accumulated in the cell nuclei. Thus, the treated cells having QDs can be tracked inside the live animal after being inoculated by virtue of their fluorescence.
Near Infrared Quantum Dots for Sentinel Lymph Node Mapping
Kim and coworkers [34] explored the utility of employing near infrared QDs emitting at 850 nm for sentinel lymph node mapping, a major procedure for the detection of roaming cancer cells in the lymph node closest to the affected organ. QDs injected intradermally into live mice were followed in real time even up to 1 cm below the skin in the sentinel lymph node. This development was a major breakthrough as the size of incision required to remove the sentinel lymph node was reduced without the use of radiolabels. Researchers are trying to use QDs for the treatment of cancer. One possibility is the irradiation of QDs by X-rays/infrared light, which would provide heat to the tumor and trigger apoptosis/programmed cell death.
Quantum Dots for Multiplexed Analysis
The ability of the QDs for multiplexed analysis of four toxins was demonstrated by Goldman and coworkers [83] using four different QDs having different emission wavelengths in a sandwich immunoassay with a single excitation source. Similarly, two spectrally different QDs were employed by Makrides and coworkers [84] for the detection of two proteins in a western blot assay. The multiplexed approach would be of extreme importance in the detection of various cancer biomarkers present at the targeted tumor site.
Self-Illuminating Quantum Dots for in vivo Imaging
Recently, it was demonstrated by Jianghong Rao’s group at Stanford University that self-illuminating QD conjugates (QD-Luc8) have potential applications for in vivo imaging [69]. The group developed an eight-mutation variant of Renilla reniformis luciferase (Luc8) that is more stable in serum and has improved catalytic efficiency. Luc8 was conjugated to polymer-coated CdSe/ZnS core shell QD 655 for making self-illuminating QD conjugates employing 1-ethyl-3-(3-dimethylaminopropyl) carbodiimide hydrochloride (EDC) crosslinker. Thus, the QD conjugates formed were self-illuminating as they luminesce by bioluminescence resonance energy transfer (BRET) in the absence of external excitation. BRET is a process in which energy is transferred non-radiatively from a light-emitting donor protein such as R. reniformis luciferase to an acceptor fluorescent protein nearby [69, 85-87]. It resulted in greatly enhanced sensitivity in small animal imaging compared to the existing QDs. One of the greatest advantages of QDs for in vivo imaging is that their emission wavelengths can be tuned throughout the near-infrared spectrum by adjusting their size, thus resulting in photostable fluorophores highly stable in biological buffers. This is due to the fact that deep tissue optical imaging is best in near-infrared spectrum as the Rayleigh scattering decreases with increasing wavelength and the major chromophores in animals i.e. hemoglobin and water have local minima in absorption in this spectrum. Antibodies against the cancer biomarkers to be detected were bound to the QD-Luc8 complex using EDC. The resulting QD-Luc8-Antibody complex formed was injected in a cancerous mouse intravenously through the tail vein for the detection of cancer biomarkers. The mouse was then anesthetized and transferred into the light tight chamber. Subsequently, after a few minutes, the substrate for Luc8 i.e. coelenterazine was injected intravenously and the in vivo bioluminescent images were taken.
Quantum Dot Based Drug Delivery System to Target Cancer
Shuming Nie and coworkers [35] modified the original CdSe QD with an impermeable coating of polymer that prevented the leaking out of highly toxic cadmium ions from the QD conjugate and provided a means to chemically attach tumor-targeting molecules and drug delivery functionality to the QD conjugate. The group is working on the development of a drug delivery system targeted to the cancer cells. It is developing QDs conjugated to peptides or antibodies to target human tumor cells growing in mice. QDs would be tuned to radiate in the infrared region to prevent tissue damage from the QDs energy emissions. QDs conjugated to peptide/antibodies specific against the cancer marker on the surface of the target cancer cells would be made to release the drug only when hit with laser light. This would allow control of the cells that will receive the toxin, thus minimizing side effects. There are also on-going efforts by the group to extend the wavelength of fluorescence of the QDs above 900 nm since there are hardly any biomolecules which emit above this wavelength.
The Current State of Play
Today with the help of QD technology, cancer researchers are capable of observing the fundamental molecular events occurring in the tumor cells. This has been made possible by tracking the QDs of different sizes and thus different colors, tagged to multiple different biomoleules, in vivo by fluorescent microscopy. QD technology holds a great potential for applications such as in nanobiotechnology and medical diagnostics where QDs could be used as labels. But still the use of QDs in humans requires extensive research to determine the long-term effects of administering QDs.
Future Applications of Quantum Dots in Cancer Diagnosis and Treatment
Researchers have started the exploration of QDs just from the last two decades. The field is still in its infancy but it has captivated scientists and engineers due to the unique optical and electronic properties of QDs. QDs have revolutionized the field of molecular imaging. The forthcoming years would see their potential applications in different fields. One of the major areas of impact is surely the intracellular imaging of live cells. The technology will provide new insights in understanding the pathophysiology of cancer, and in imaging and screening tumors. QDs will definitely be one of the components of the envisioned multifunctional nanodevices that can detect diseased tissue, provide treatment and report progress in real time.
|
1. Chan W.C.W., Maxwell D.J., Gao X., Bailey R.E., Han M. and Nie S., “Luminescent QDs for multiplexed biological detection and imaging”, Curr. Opin. Biotechnol., 13, 40-46, 2002.
2. Alivisatos A.P., “Semiconductor clusters, nanocrystals, and quantum dots”, Science, 271, 933-937, 1996.
3. Han M., Gao X., Su J.Z. and Nie S., “Quantum dot-tagged microbeads for multiplexed optical coding of biomolecules”, Nat. Biotechnol., 19, 631-635, 2001.
4. Niemeyer C.M., “Nanoparticles, proteins, and nucleic acids: Biotechnology meets materials science”, Angrew. Chem. Int. Ed. Engl., 40, 4128-4158, 2001.
5. Leatherdale C.A., Woo W.K., Mikulec F.V. and Bawendi M.G., “On the absorption cross section of CdSe nanocrystal quantum dots”, J. Phys. Chem. B, 106, 7619-7622, 2002.
6. Bruchez M., Moronne M., Gin P., Weiss S. and Alivisatos A.P., “Semiconductor nanocrystals as fluorescent biological labels”, Science, 281, 2013-2015, 1998.
7. Mattoussi H., Mauro J.M., Goldman E.R., Anderson G.P., Sundar V.C., Mikulec F.V. and Bawendi M.G., “Self-assembly of CdSe-ZnS quantum dot bioconjugates using an engineered recombinant protein”, J. Am. Chem. Soc., 122, 12142-12150, 2000.
8. Dubertret B., Skourides P., Norris D.J., Noireaux V., Brivanlou A.H. and Libchaber A., “In vivo imaging of QDs encapsulated in phospholipid micelles”, Science, 298, 1759-1762, 2002.
9. Jaiswal J.K., Mattoussi H., Mauro J.M. and Simon S.M., “Long-term multiple color imaging of live cells using quantum dot bioconjugates”, Nat. Biotechnol., 21, 47-51, 2003.
10. Larson D.R., Zipfel W.R., Williams R.M., Clark S.W., Bruchez M.P., Wise F.W. and Webb W.W., “Water-soluble quantum dots for multiphoton fluorescence imaging in vivo”, Science, 300, 1434-1436, 2003.
11. Ishii, D., Kinbara K., Ishida Y., Ishii N., Okochi M., Yohda M. and Aida T., “Chaperonin-mediated stabilization and ATP-triggered release of semiconductor nanoparticles”, Nature, 423, 628-632, 2003.
12. Medintz I.L., Clapp A.R., Mattoussi H., Goldman E.R., Fisher B. and Mauro J.M., “Self-assembled nanoscale biosensors based on quantum dot FRET donors”, Nat. Mater., 2, 630-639, 2003.
13. Dahan M., Levi S., Luccardini C., Rostaing P., Riveau B. and Triller A., “Diffusion dynamics of glycine receptors revealed by single-quantum dot tracking”, Science, 302, 442-445, 2003.
14. Rosenthal S.J., Tomlinson I., Adkins E.M., Schroeter S., Adams S., Swafford L., McBride J., Wang Y., DeFelice L.J. and Blakely R.D., “Targeting cell surface receptors with ligand-conjugated nanocrystals”, J. Am. Chem. Soc., 124, 4586-4594, 2002.
15. Mahtab R., Harden H.H. and Murphy C.J., “Temperature- and salt-dependent binding of long DNA to protein-sized quantum dots: thermodynamics of “inorganic protein”-DNA interactions”, J. Am. Chem. Soc., 122, 14-17, 2000.
16. Sun B., Xie W., Yi G., Chen D., Zhou Y. and Cheng J., “Microminiaturized immunoassays using quantum dots as fluorescent label by laser confocal scanning fluorescence detection”, J. Immunological Methods, 249, 85-89, 2001.
17. Pathak S., Choi S.-K., Arnheim N. and Thompson M.E., “Hydroxylated quantum dots as luminescent probes for in situ hybridization”, J. Am. Chem. Soc., 123, 4103-4104, 2001.
18. Klarreich E., “Biologists join the dots”, Nature, 413, 450-452, 2001.
19. Mitchell P., “Turning the spotlight on cellular imaging”, Nat. Biotechnol., 19, 1013-1017, 2001.
20. Jovin T.M., “Quantum dots finally come of age”, Nat. Biotechnol., 21, 32-33, 2003.
21. Seydel C., “Quantum dots get wet”, Science, 3000, 80-81, 2003.
22. Taton T.A., “Bio-nanotechnology: two way traffic”, Nat. Mater., 2, 73-74, 2003.
23. Bentolila L.A. and Weiss S., “Biological quantum dots go live”, Phys. World, 16, 23-24, 2003.
24. Uren R.F., “Cancer surgery joins the dots”, Nat. Biotechnol., 22, 38-39, 2004.
25. Michalet X., Pinaud F. , Lacoste T.D., Dahan M., Bruchez M.P., Alivisatos A.P. and Weiss S., “Properties of fluorescent semiconductor nanocrystals and their application to biological labelling”, Single Mol., 2, 261-276, 2001.
26. Sutherland A.J., “Quantum dots as luminescent probes in biological systems”, Curr. Opin. Solid State Mater. Sci., 6, 365-370, 2003.
27. Watson A., Wu X. and Bruchez M., “Lighting up cells with quantum dots”, Biotechniques, 34, 296-303, 2003.
28. Parak W.J., Gerion D., Pellegrino T., Zanchet D., Micheel C., Williams S.C., Boudreau R., Le Gros M.A., Larabell C.A. and Alivisatos A.P., “Biological applications of colloidal nanocrystals”, Nanotechnology, 14, R15-27, 2003.
29. Bagwe R.P., Zhao X. and Tan W., “Bioconjugated luminescent nanoparticles for biological applications”, J. Dispersion. Sci. Technol., 24, 453-464, 2003.
30. Dubertret B., “In vivo imaging using quantum dots”, J. Med. Sci., 19, 532-534, 2003.
31. Alivisatos A.P., “The use of nanocrystals in biological detection”, Nat. Biotechnol., 22, 47-51, 2004.
32. Pellegrino T., Kudera S., Liedl T., Javier A.M., Manna L. and Parak W.J., “On the development of colloidal nanoparticles towards multifunctional structures and their possible use for biological applications”, Small, 1, 48-63, 2005.
33. Michalet X., Pinaud F.F., Bentolila L.A., Tsay J.M., Doose S., Li J.J., Sundaresan G., Wu A.M., Gambhir S.S. and Weiss S., “Quantum dots for live cells, in vivo imaging, and diagnostics”, Science, 307, 538-544, 2005.
34. Kim S., Lim Y.T., Soltesz E.G., De Grand A.M., Lee J., Nakayama A., Parker J.A., Mihaljevic T., Laurence R.G., Dor D.M., Cohn L.H., Bawendi M.G. and Frangioni J.V., “Near-infrared fluorescent type II quantum dots for sentinel lymph node mapping”, Nat. Biotechnol., 22, 93-97, 2004.
35. Gao X., Cui Y., Levenson R.M., Chung L.W.K. and Nie S., “In vivo cancer targeting and imaging with semiconductor quantum dots”, Nat. Biotechnol., 22, 969-976, 2004.
36. Jaiswal J.K. and Simon S.M., “Potentials and pitfalls of fluorescent quantum dots for biological imaging”, Trends Cell Biol., 14, 497-504, 2004.
37. Medintz I.L., Uyeda H.T., Goldman E.R. and Mattoussi H., “Quantum dot bioconjugates for imaging, labelling and sensing”, Nat. Mater., 4, 435-446, 2005.
38. Wu X., Liu H., Haley K.N., Treadway J.A., Larson J.P., Ge N., Peale F. and Bruchez M.P., “Immunofluorescent labeling of cancer marker Her2 and other cellular targets with semiconductor quantum dots”, Nature Biotechnology, 21, 41-46, 2003.
39. Mattheakis L.C., Dias J.M., Choi Y.-J., Gong J., Bruchez M., Liu J. and Wang E., “Optical coding of mammialian cells using semiconductor quantum dots”, Anal. Biochem., 327, 200-208, 2004.
40. Rosenthal S.J., “Bar-coding biomolecules with fluorescent nanocrystals”, Nat. Biotechnol., 19, 621-622, 2001.
41. Talapin D.V., Rogach A.L., Kornowski A., Haase M. and Weller H., “Highly luminescent monodisperse CdSe and CdSe/ZnS nanocrystals synthesized in a hexadecylamine-trioctylphosphine oxide-trioctylphosphine mixture”, Nano Lett., 1, 207-211, 2001.
42. Peng Z.A. and Peng X., “Formation of high-quality CdTe, CdSe, and CdS nanocrystals using CdO as precursor”, J. Am. Chem. Soc., 123, 183-184, 2001.
43. Reiss P., Bleuse J. and Pron A., “Highly luminescent CdSe/ZnSe core/shell nanocrystals of low size dispersion”, Nano Lett., 2, 781-784, 2002.
44. Parak W.J., Gerion D., Zanchet D., Woerz A. S., Pellegrino T., Micheel C., Williams S. C., Seitz M., Bruehl R. E., Bryant Z., Bustamante C., Bertozzi C. R. and Alivisatos A. P., “Conjugation of DNA to silanized colloidal semiconductor nanocrystaline quantum dots”, Chem. Mater., 14, 2113-2119, 2002.
45. Wilhelm C., Billotey C., Roger J., Pons J.N., Bacri J.C. and Gazeau F., “Intracellular uptake of anionic superparamagnetic nanoparticles as a function of their surface coating”, Biomaterials, 24, 1001-1011, 2003.
46. Pellegrino T., Manna L. and Kudera S., “Hydrophobic nanocrystals coated with an amphiphilic polymer shell: a general route to water soluble nanocrystals”, Nano Lett., 4, 703-07, 2004.
47. Petruska M.A., Bartko A.P. and Klimov V.I., “An amphiphilic approach to nanocrystal quantum dot-titania nanocomposites”, J. Am. Chem. Soc., 126, 714-715, 2004.
48. Ballou B., Lagerholm B.C., Ernst L.A., Bruchez M.P. and Waggoner A.S., “Noninvasive imaging of quantum dots in mice”, Bioconjug. Chem., 15, 79-86, 2004.
49. Murray C.B., Kagan C.R. and Bawendi M.G., “Synthesis and characterization of monodisperse nanocrystals and close-packed nanocrystal assemblies”, Annu. Rev. Mater. Sci., 30, 545-610, 2000.
50. Qu L. and Peng X., “Control of photoluminescence properties of CdSe nanocrystals in growth”, J. Am. Chem. Soc., 124, 2049-2055, 2002.
51. Kippeny T., Swafford L.A. and Rosenthal S.J., “Semiconductor nanocrystals: a powerful visual aid for introducing the particle in a box”, J. Chem Educ., 79, 1094, 2002.
52. Yu W.W., Qu L., Guo W. and Peng X., “Experimental determination of the extinction coefficient of CdTe, CdSe, and CdS nanocrystals”, Chem. Mater., 15, 2854-2860, 2003.
53. Gao X.H. and Nie S.M., “Molecular profiling of single cells and tissue specimens with quantum dots”, Trends Biotechnol., 21, 371-373, 2003.
54. Jovin T.M., “Quantum dots finally come of age”, Nat. Biotechnol., 21, 32-33, 2003.
55. Lidke D.S., Nagy P., Heintzmann R., Arndt-Jovin D.J., Post J.N., Grecco H.E., Jares-Erijman E.A. and Jovin T.M., “Quantum dot ligands provide new insights into erbB/HER receptor-mediated signal transduction”, Nat. Biotechnol., 22, 198-203, 2004.
56. Derfus A.M., Chan W.C.W. and Bhatia S.N., “Probing the cytotoxicity of semiconductor quantum dots”, Nano Lett., 4, 11-18, 2004.
57. Akerman M.E., Chan W.C.W., Laakkonen P., Bhatia S.N. and Ruoslahti, E., “Nanocrystal targeting in vivo”, PNAS, 99, 12617-21, 2002.
58. Ness J.M., Akhtar R.S., Latham C.B. and Roth K.A., “Combined tyramide signal amplification and quantum dots for sensitive and photostable immunofluorescence detection”, J. Histochem. Cytochem., 51, 981-987, 2003.
59. Peng X., Schlamp M.C., Kadavanich, A.V. and Alivisatos A.P., “Epitaxial growth of highly luminescent CdSe/CdS core/shell nanocrystals with photostability and electronic accessibility”, J. Am. Chem. Soc., 119, 7019-7029, 1997.
60. Murray C.B., Norris D.J. and Bawendi M.G., “Synthesis and characterization of nearly monodisperse CdE (E=S, Se, Te) semiconductor nanocrystallites”, J. Am. Chem. Soc., 115, 8706-8715, 1993.
61. Dabbousi B.O., Rodriguez-Viejo J., Mikulec F.V., Heine J.R., Mattoussi H., Ober R., Jensen K.F. and Bawendi M.G., “(CdSe)ZnS core-shell quantum dots: Synthesis and characterization of a size series of highly luminescent nanocrystallites”, J. Phys. Chem. B, 101, 9463-9475, 1997.
62. Hines M.A. and Guyot-Sionnest P., “Synthesis of strongly luminescing ZnS-capped CdSe nanocrystals”, J. Phys. Chem. B, 100, 468-471, 1996.
63. Chan W.C.W. and Nie S., “Quantum dot bioconjugates for ultrasensitive nonisotopic detection”, Science, 281, 2016-2018, 1998.
64. Mitchell G.P., Mirkin C.A. and Letsinger R.L., “Programmed assembly of DNA functionalized quantum dots”, J. Am. Chem. Soc., 121, 8122-8123, 1999.
65. Nirmal M., Dabbousi B.O., Bawendi M.G., Macklin J.J., Trautman J.K., Harris T.D. and Brus L.E., “Fluorescence intermittency in single cadmium selenide nanocrystals”, Nature, 383, 802-804, 1996.
66. Efros, A.L. and Rosen, M., “Random telegraph signal in the photoluminescence intensity of a single quantum dot”, Phys. Rev. Lett., 78, 1110-1113, 1997.
67. Chen Y. and Rosenzweig Z., “Luminescent CdS quantum dots as selective ion probes”, Anal. Chem., 74, 5132-5138, 2002.
68. Gao X., Cui Y., Levenson R.M., Chung L.W.K. and Nie, S., “In vivo cancer targeting and imaging with semiconductor quantum dots”, Nature Biotechnology, 22, 969-76, 2004.
69. So M.K., Xu C., Loening A.M., Gambhir S.S. and Rao J., “Self-illuminating quantum dot conjugates for in vivo imaging”, Nature Biotechnology, 24, 339-43, 2006.
70. Duncan R., “The dawning era of polymer therapeutics”, Nat. Rev. Drug Discov., 2, 347-360, 2003.
71. Jain R.K., “Transport of molecules, particles, and cells in solid tumors”, Ann. Rev. Biomed. Eng., 1, 241-263, 1999.
72. Jain R.K., “Delivery of molecular medicine to solid tumors: lessons from in vivo imaging of gene expression and function”, J. Control. Release, 74, 7-25, 2001.
73. Cheong W.F., Prahl S.A. and Welch A.J., “A review of the optical properties of biological tissues”, IEEE J. Quantum Electron., 26, 2166-2185, 1990.
74. Ntziachristos V., Bremer C. and Weissleder R., “Fluorescence imaging with near-infrared light: new technological advances that enable in vivo molecular imaging”, Eur. Radiol., 13, 195-208, 2003.
75. Zhang C.Y., Ma H., Nie S.M., Ding Y., Jin L. and Chen D.Y., “Quantum dot-labeled trichosanthin”, Analyst, 125, 1029-1031, 2000.
76. Winter J.O., Liu T.Y., Korgel B.A. and Schmidt C.E., “Recognition molecule directed interfacing between semiconductor quantum dots and nerve cells”, Adv. Mater., 13, 1673-1677, 2001.
77. Kloepfer J.A., Mielke R.E., Wong M.S., Nealson K.H., Stucky G. and Nadeau J.L., “Quantum dots as strain- and metabolism-specific microbiological labels”, Appl. Environ. Microbiol., 69, 4205-4213, 2003.
78. Goldman E.R., Anderson, G.P., Tran P.T., Matttoussi H., Charles P.T. and Mauro J.M., “Conjugation of luminescent quantum dots with antibodies using an engineered adaptor protein to provide new reagents for fluoroimmunoassays”, Anal. Chem., 74, 841-47, 2002.
79. Kapanidis A.N., Ebright Y.W. and Ebright R.H., “Site-specific incorporation of fluorescent probes into protein: hexahistidine-tag-mediated fluorescent labeling with (Ni(2+):nitrilotriacetic Acid (n)-fluorochrome conjugates”, J. Am. Chem. Soc., 123, 12123-25, 2001.
80. Tokumasu F. and Dvorak J., “Development and application of quantum dots for immunocytochemistry of human erythrocytes”, J. Microsc., 211, 256-261, 2003.
81. Gerion D., Chen F., Kannan B., Fu A., Parak W.J., Chen D.J., Majumdar A. and Alivisatos A.P., “Ultra-fast room-temperature single nucleotide polymorphism detection and multi-allele DNA detection using fluorescent nanocrystal probes and microarray”, Anal. Chem., 75, 4766-4772, 2003.
82. Dahan M., Laurence T., Pinaud F., Chemla D. S., Alivisatos A. P., Sauer M. and Weiss S., “Time-gated biological imaging by use of colloidal quantum dots”, Opt. Lett., 26, 825-827, 2001.
83. Goldmann E.R., Clapp A.R., Anderson G.P., Uyeda H.T., Mauro J.M., Medintz I.L. and Mattoussi H., “Multiplexed toxin analysis using four colors of quantum dot fluororeagents”, Anal. Chem., 76, 684-88, 2004.
84. Makrides S.C., Gasbarro C. and Bello J.M., “Bioconjugation of quantum dot luminescent probes for western blot analysis”, Biotechniques, 39, 501-506, 2005.
85. Ward W.W. and Cormier M.J., “Energy transfer via protein-protein interaction in Renilla bioluminescence”, Photochem. Photobiol., 27, 389-396, 1978.
86. Wilson T. and Hastings J.W., “Bioluminescence”, Annu. Rev. Cell Dev. Biol., 14, 197-230, 1998.
87. De A. and Gambhir S.S., “Non-invasive imaging of protein-protein interactions from live cells and living subjects using bioluminescence resonance energy transfer”, FASEB J., 19, 2017-2019, 2005.
|