By Nidhi DhullReviewed by Lexie CornerUpdated on Aug 2 2024
Stanene, a single layer of tin atoms with a buckling honeycomb structure, was first proposed as a large gap quantum spin Hall (QSH) insulator in 2013.1,2 Its tunable band structure and topological characteristics, achieved by varying substrates, chemical modifications, and layer thickness, have the potential to make it the world's first electrical conductor with 100 % efficiency.2
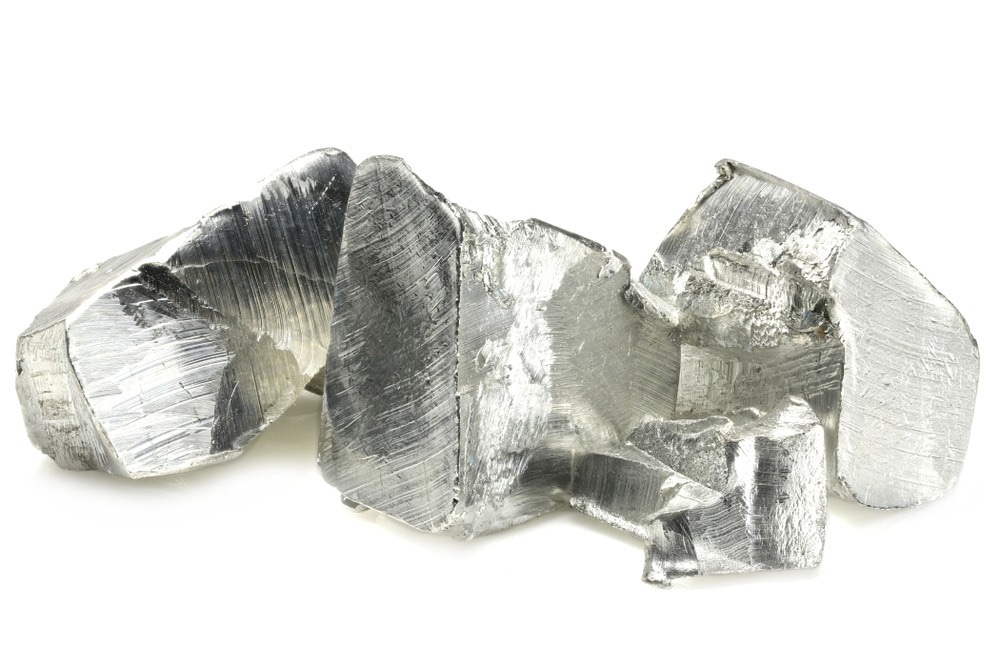
Image Credit: Bjoern Wylezich/Shutterstock.com
In contrast to graphene, electrons in stanene travel along the lattice edge without collisions at room temperature. Recent successes in realizing stable and free-standing stanene heterostructures have expanded its potential applications.3 This article explores the basic properties of stanene, its applicability in modern devices, and existing challenges.
Properties and Structure of Stanene?
Stanene is a mono-elemental layer of tin atoms with a structure similar to graphene’s honeycomb lattice.3 However, unlike graphene (sp2-type hybridization), stanene has a buckled hexagonal lattice crystal structure (mixed sp2-sp3 hybridization). Consequently, stanene has two structural forms: low-buckled stanene and a dumbbell form, where the former has shorter lattice and buckling parameters.1
Notably, stanene possesses dangling bonds on edges, allowing easy chemical passivation and a more stable sp3 configuration.2 Thus, stanene is regarded as a single layer of α-Sn (a gray allotrope of tin with a less dense diamond cubic structure).2,3 The spatial distribution of edge states observed in recent scanning tunneling microscope analyses has evidenced its QSH property.2
Computational studies have revealed its anisotropic mechanical properties, exhibiting different values of Young's modulus, ultimate tensile strength, and Poisson's ratio in different edge directions.1,3 Additionally, the tensile strength and elastic modulus decrease with increasing defects such as single vacancy, double vacancy, and Stone-Wales defects.1
How Does Stanene Work?
Two-dimensional (2D) topological insulators (TIs), called QSH insulators, exhibit an insulating gap in the bulk and conducting channels at edges without backscattering. Additionally, each edge provides a quantized conductance of 2e2/h. These conducting channels, free of power dissipation, can allow the flow of electrons with 100 % efficiency.2
Stanene was first proposed as a QSH insulator with a gap of ∼0.1 eV in 2013 due to its freestanding behavior and possible chemical functionalization. However, enhanced spin-orbit coupling effects (SOC) in stanene are responsible for its insulating nature. Moreover, its dangling bonds offer additional degrees of freedom to tune the band structure and topological properties.2
The electron and phonon transport in stanene governs its thermal characteristics. Without the SOC effect, the massless Dirac fermions in stanene are governed by the modified Wiedemann-Franz law of electron thermal conductivity. Consequently, its electron thermal conductivity is much higher than the phonon thermal conductivity.1
Despite no superconductivity observed in bulk α-Sn, few-layer stanene exhibits superconductivity in transport measurements, making it a probable topological superconductor. Decorated stanene, such as SnI, can show the Rashba SOC effect if the inversion symmetry is broken. This can be achieved by doping, applying an external electric field, or forming heterostructures.2
Advantages and Applications of Stanene
Stanene’s graphene-like nature, high functionalization, and defect engineering capabilities make it suitable for various applications.1 Its tunable buckling parameter through substrate interfacial strain, electric field, doping, and chemical functionalization provides essential functionality for application in quantum electronics with dissipation-less conduction.3
Epitaxially grown stanene on InSb substrate exhibits a stable buckled 2D monolayer structure with a large spin-orbit gap of 0.44 eV, ideal for electronic QSH applications at room or higher temperatures. This large nontrivial bandgap of stanene is highly promising for future applications in nanoelectronics, spintronics, and quantum computing, potentially replacing silicon.3
Stanene's buckled structure and high chemical reactivity enables its use in sensing applications. The adsorption of various metallic ions and organic/inorganic gas molecules on stanene alters its electronic structures due to geometrical strain, which can be exploited to fabricate sensing devices.3
Theoretical studies have predicted the suitability of stanene-based 2D nanomaterials as efficient anode materials for sodium-ion batteries and membranes for hydrogen/helium gaseous phase purification systems. This is attributed to stanene’s structural robustness and tunable pore distribution in the honeycomb lattice.3
Stanene is also well-suited as a catalyst in nitrogen and CO2 reduction reactions, which are fundamental to hydrogen energy production and application.1
Bilayer structures of stanene with graphene, ZnO, and SnF exhibit enhanced photo-responses from the ultraviolet to visible spectrum range, attributable to the optimized work functions and electronic states in these bilayer systems. Thus, they are promising for photonic and optoelectronic applications such as photovoltaics and optical sensors.3
Challenges and Limitations of Stanene
Despite its promising properties, stanene's practical application faces challenges related to synthesis, stability, and device integration.1 The primary issue is the lack of experimental evidence to support theoretical predictions.2
Stanene has been studied for its QSH insulation and topological superconductivity, but firm conclusions are lacking. For instance, while stanene exhibits QSH insulation on Cu and Bi substrates, these metallic substrates hinder further transport measurements of the quantized edge conducting channels.2 Additionally, the heavy Sn atoms interact with metallic substrates to form surface alloys, inhibiting the exotic quantum behavior of stanene.3
Scalable and controlled production of high-quality stanene is limited by the poor yields, irregular morphology, and contamination issues of current technologies, hampering the device’s performance and repeatability.1
Although theoretical calculations demonstrate stanene’s stability, fabricating high-quality stanene films is difficult. Molecular beam epitaxy (MBE) is mostly used for such films, but finding a suitable substrate for MBE growth with a small lattice mismatch is challenging. An incorrect substrate lattice results in the formation of β-Sn or amorphous Sn.2
Stanene: Future Prospects
Stanene is a promising material that can revolutionize the semiconductor industry. Significant efforts are being made to exploit its properties in various applications. For instance, a recent study in PHYSICSAccess demonstrated the optoelectronic potential of stanene and stanene doped with non-metals like phosphorous and sulfur. This doping changed the zero band gap of stanene into a direct band gap and increased its refractive index.4
Another recent study in Physical Chemistry Chemical Physics proposed using armchair stanene nanoribbons (ASnNRs) for the early detection of lung cancer. The effect of a uniform external electric field was analyzed on the sensing behavior of ASnNRs. Applying an electric field influenced the adsorption energy and charge transfer across stanene, enabling enhanced sensitivity and selectivity towards lung cancer biomarkers.5
Stanene’s compatibility with silicon technology holds tremendous future opportunities in nanoelectronic devices. Experimental evidence on its topological properties will accelerate its adoption in advanced technologies.
More from AZoNano: What is an Atomic Force Microscope?
References and Further Reading
1. Santra, S., Ghosh, A., Das, B., Pal, S., Pal, S., Adalder, A. (2024). Beyond the horizons of graphene: xenes for energy applications. RSC Sustainability. DOI: 10.1039/D3SU00445G. https://pubs.rsc.org/en/content/articlelanding/2024/su/d3su00445g
2. Zhao, C.-X., Jia, J.-F. (2020). Stanene: A good platform for topological insulator and topological superconductor. Frontiers of Physics. DOI: 10.1007/s11467-020-0965-5. https://link.springer.com/article/10.1007/s11467-020-0965-5
3. Sahoo, SK., Wei, K. (2019). A Perspective on Recent Advances in 2D Stanene Nanosheets. Advanced Materials Interfaces. DOI: 10.1002/admi.201900752. https://onlinelibrary.wiley.com/doi/full/10.1002/admi.201900752
4. Gidado, A., Abubakar, L., Taura, L., Lawal, A., Isah, A. (2024). Structural, Electronic, and Optical Properties of Stanene and Stanene-Doped Non-metals for Optoelectronics Applications: A first-principle Study. PHYSICSAccess. DOI: 10.47514/phyaccess.2024.4.1.002. https://physicsaccess.com/articles/published/PA-JPET-Vol%204-Issue%201_161.pdf
5. Mashhadbani, M., Faizabadi, E. (2023). Enhanced sensing performance of armchair stanene nanoribbons for lung cancer early detection using an electric field. Physical Chemistry Chemical Physics. DOI: 10.1039/D3CP04281B. https://pubs.rsc.org/en/content/articlelanding/2023/cp/d3cp04281b
Disclaimer: The views expressed here are those of the author expressed in their private capacity and do not necessarily represent the views of AZoM.com Limited T/A AZoNetwork the owner and operator of this website. This disclaimer forms part of the Terms and conditions of use of this website.