Pomelo is one of the world’s most popular fruits., with China contributing to the world's largest supply volume of pomelo production. As a result, the fruit has a significant economic influence in China.
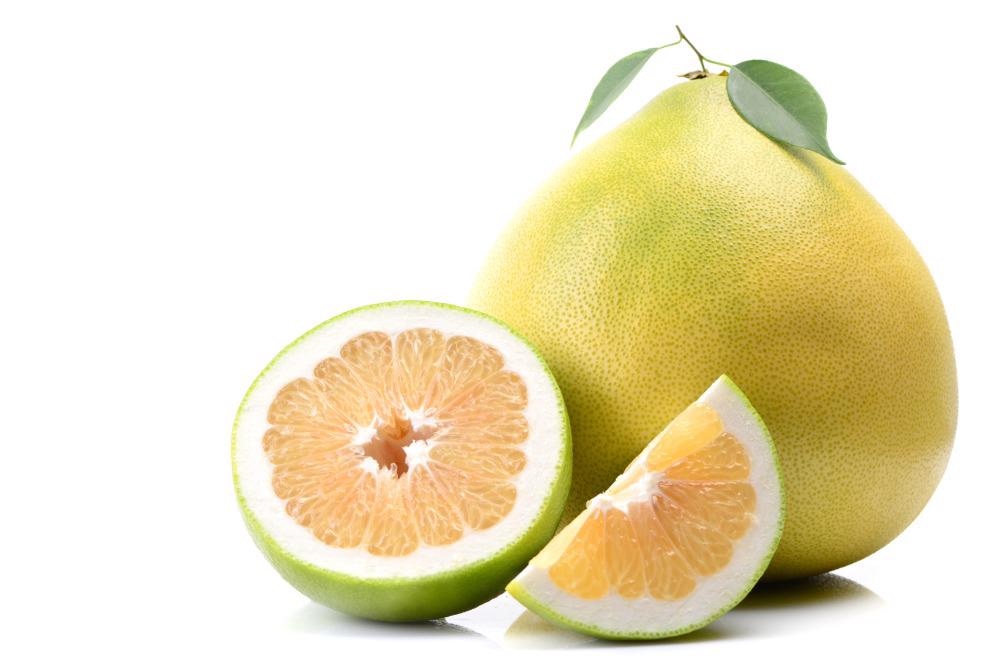
Image Credit: Spayder pauk_79/Shutterstock.com
Pomelo peels make up roughly 45% of the overall weight of the fruit and are frequently discarded as agricultural trash. This trash has an environmental impact and pollutes the environment; thus, it must be carefully controlled. Pomelo peel is also a potential carbon precursor material since it contains cellulose, hemicellulose, and lignin.
As a result, several procedures and temperature regulations have been explored by researchers to convert pomelo peels into more desirable materials, such as highly ordered and conductive graphitized materials. The team published their research in the MDPI journal crystals.
The arc discharge technique, laser vaporization, and plasma and thermal chemical vapor deposition are all examples of generic graphitization procedures. However, these approaches cannot be extensively employed due to several constraints leading to a research focus on simplifying the method.
Different synthesis routes are used in this research to lower the temperature of graphitized carbon materials and transform waste pomelo peels into highly graphitized materials. The synthesis effect of conventional approaches and the new one proposed by the team were compared. Following the team's improvements, a high degree of graphitization effect could be accomplished at a reduced temperature.
Methodology
Two procedures were used to treat discarded pomelo peels (Figure 1). The pomelo peels were washed twice, once with tap water and again with deionized water. They were then dried for 24 hours at 80° C in an oven, and crushed to produce powders. Those less than 147 µm in size were chosen to produce a generally homogenous fine powder.
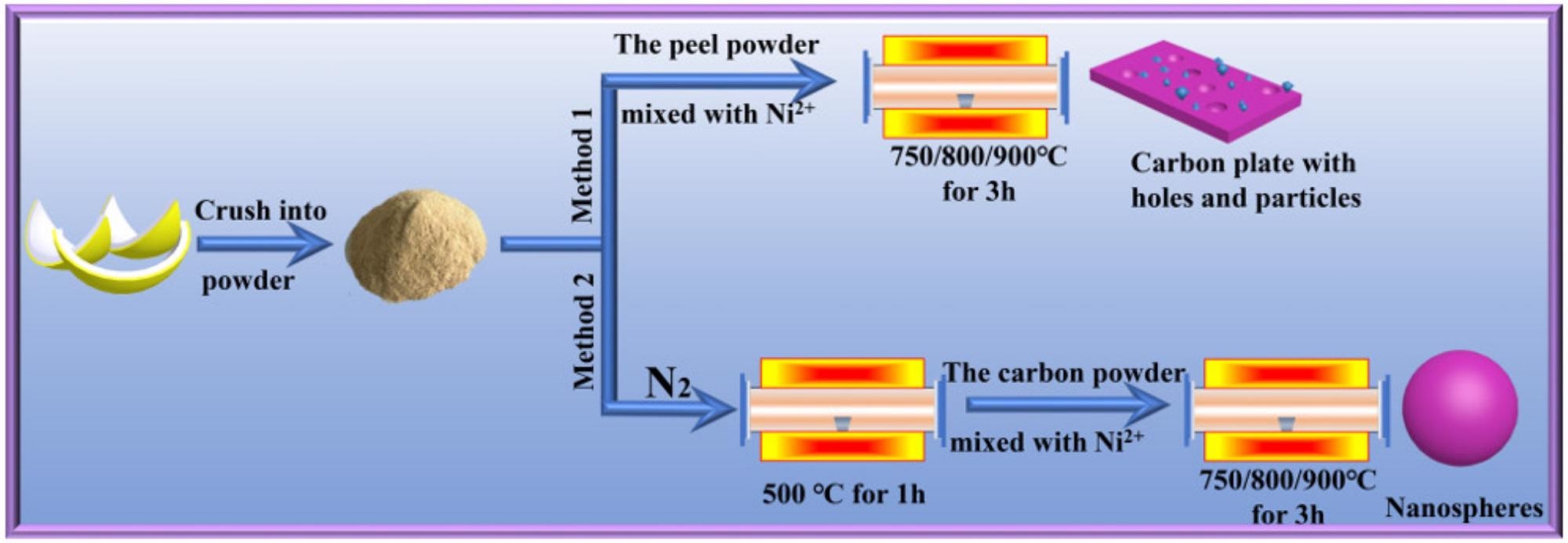
Figure 1. Schematic flow diagram of the traditional method and the improved method. Image Credit: Zeng, et al., 2022
The materials were analyzed using the Bruker D8 equipment for X-ray powder diffraction (XRD). The surface chemistry and chemical state of sample elements were investigated using X-ray photoelectron spectroscopy (XPS). Thermo Scientific was used to do the XPS.
To examine the degree of order of carbon structure, Raman spectra were collected in a Raman microscope (Via Reflex) with a laser excitation wavelength of 532 nm. The data acquisition duration was 10 seconds, and the scanning range was 500–3500 cm-1. To confirm the correctness of the data, each sample was examined three times.
Peak deconvolution was used to analyze Raman spectra using Peakfit software. A spectrometer was used to analyze the changes in functional groups during graphitization using Fourier transform infrared (FTIR) spectroscopy.
Lattice fringes were observed using HRTEM. To ensure homogeneous dispersion, all samples for HRTEM analysis were distributed in ethanol and sonicated for 10 minutes. They were then deposited onto carbon-coated grids and dried at ambient temperature.
This was used to detect sample images and lattice fringes. On the FEI Talos F200S, SAED was employed to verify the degree of graphitization.
Results and Discussion
The XRD spectrum was used to determine the degree of graphitization of the samples. Figure 2a shows that the corresponding (002), (100), (101), (004), and (110) diffraction peaks have apparent strong peaks at ca. 26°, 43°, 54°, 78°, and 83°.
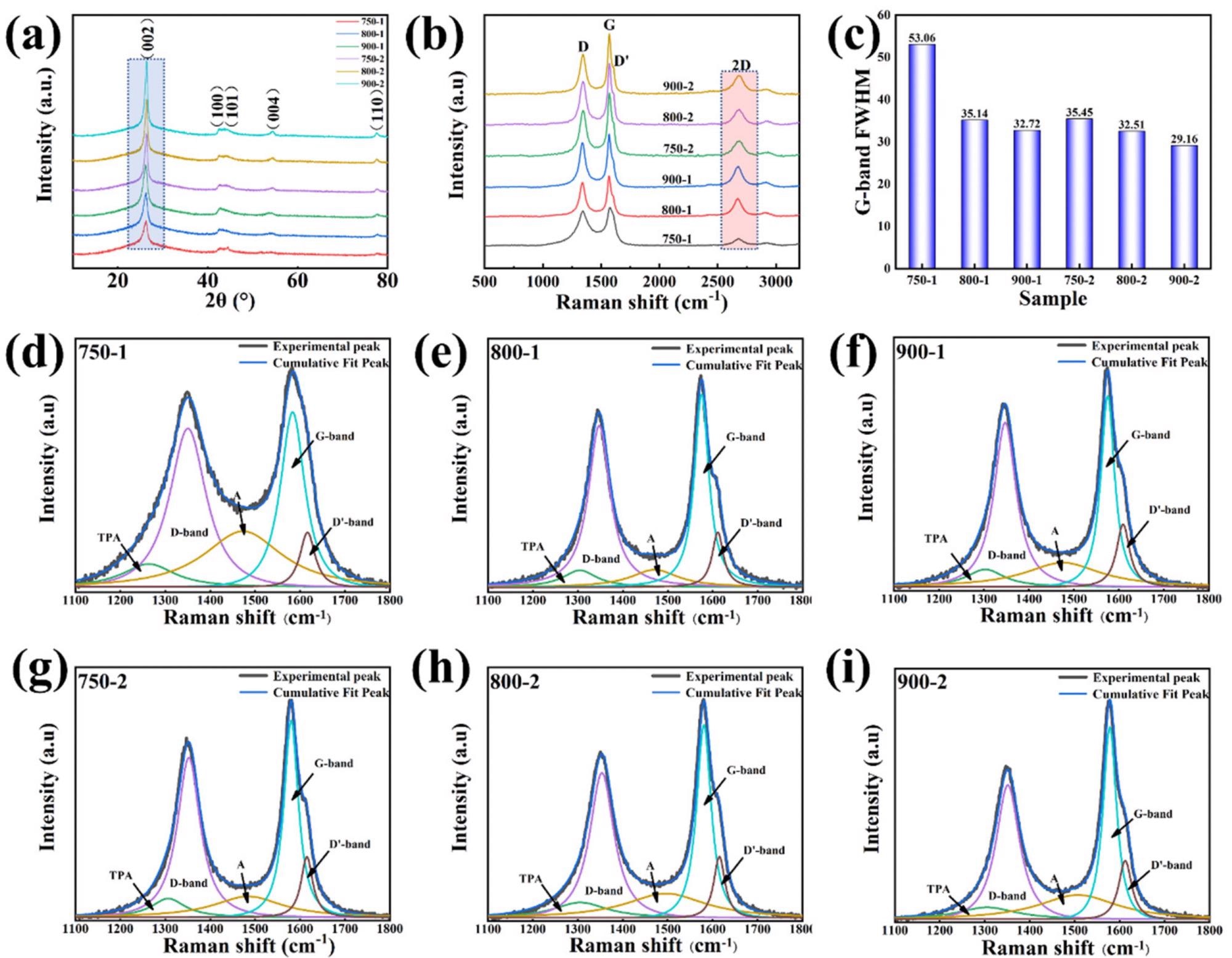
Figure 2. (a) XRD patterns of the graphitized pomelo peel; (b) Raman of the graphitized pomelo peel; (c) FWHM of G-band; Deconvolution of Raman spectra for (d) 750-1, (e) 800-1, (f) 900-1, (g) 750-2, (h) 800-2, (i) 900-2. Image Credit: Zeng, et al., 2022
The structural characteristics of graphitic carbon were estimated using the XRD spectrum (Figure 2a).
To compute the diffraction peak of (002), the Bragg equation was used to obtain the plane spacing d(002) as shown in Table 1.
Table 1. Structural parameters were analyzed from the curve of the XRD and Raman spectrum. Source: Zeng, et al., 2022
Sample |
d002 (nm) |
G (%) |
IG/ID |
ID/(ID + IG + ID’) |
750-1 |
0.3423 |
19.77 |
1.10 |
0.701 |
800-1 |
0.341 |
34.88 |
1.17 |
0.655 |
900-1 |
0.3398 |
48.84 |
1.19 |
0.641 |
750-2 |
0.3376 |
74.42 |
1.33 |
0.618 |
800-2 |
0.3375 |
75.58 |
1.37 |
0.591 |
900-2 |
0.3371 |
80.23 |
1.43 |
0.509 |
Figure 2b shows a Raman spectroscopy graph, which is used to investigate the degree of order in samples. The Raman spectra of samples in the range of 1100–1800 cm-1 were created using a Gauss–Lorentzian curve fitting algorithm, as shown in Figure 2d–i. Figure 2c summarizes the output of the FWHM analysis of the peak-fitted G-band.
The FWHM of the G-band drops steadily as temperature rises, as illustrated in Figure 2c.
A higher degree of order is represented by a higher IG/ID value. The IG/ID values of the 750-1, 800-1, and 900-1 samples are 1.10, 1.17, and 1.19, respectively, as shown in Table 1, suggesting the degree of order of the carbon structure to grow progressively as temperature rises.
The functional groups of the material are reflected in FTIR spectral analysis (Figure 3a).
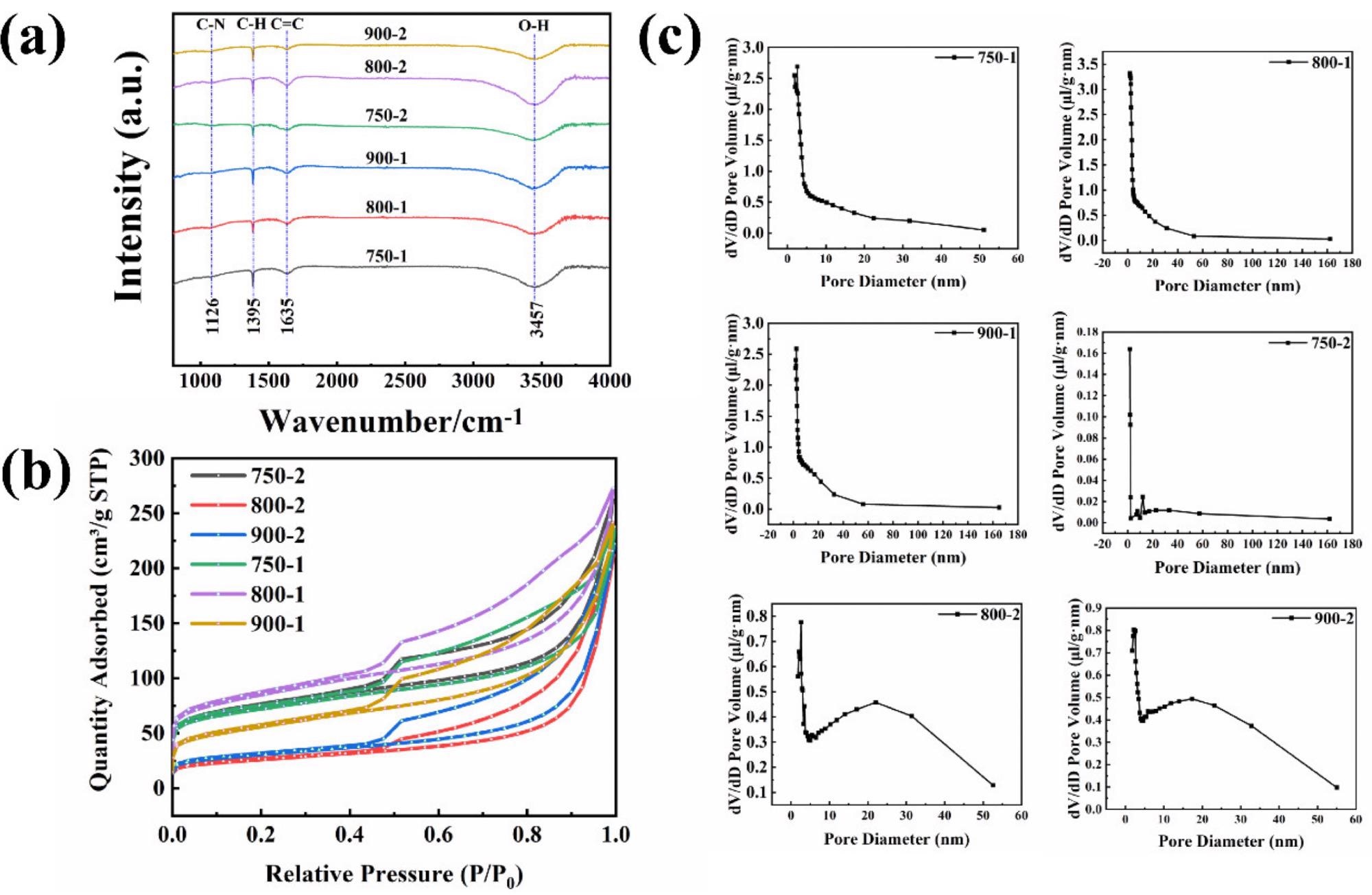
Figure 3. (a) FTIR of the graphitized peel; (b) N2 adsorption–desorption isotherms for the graphitized peel; (c) Pore size distribution image of the graphitized peel. Image Credit: Zeng, et al., 2022
The isotherms of the samples, as shown in Figure 3b, adhere to the typical integral of the IV isotherms. Figure 3c shows the pore size distribution, and the presence of micropores, mesopores, and macropores in the sample agrees with the data in Figure 3b.
750-2, 800-2, and 900-2 looked spheroidized after treatment with procedure two (Figure 4a–c). High-magnification characterization was used to examine the morphology of the pomelo peel after treatment with method two (Figure 4d–f).
The diameter of the nanospheres steadily reduces as the temperature rises, as seen in Figure 4g–i, with the diameters ranging from 750-2, 800-2, and 900-2 being 213.23 nm, 205.32 nm, and 176.8 nm, respectively.
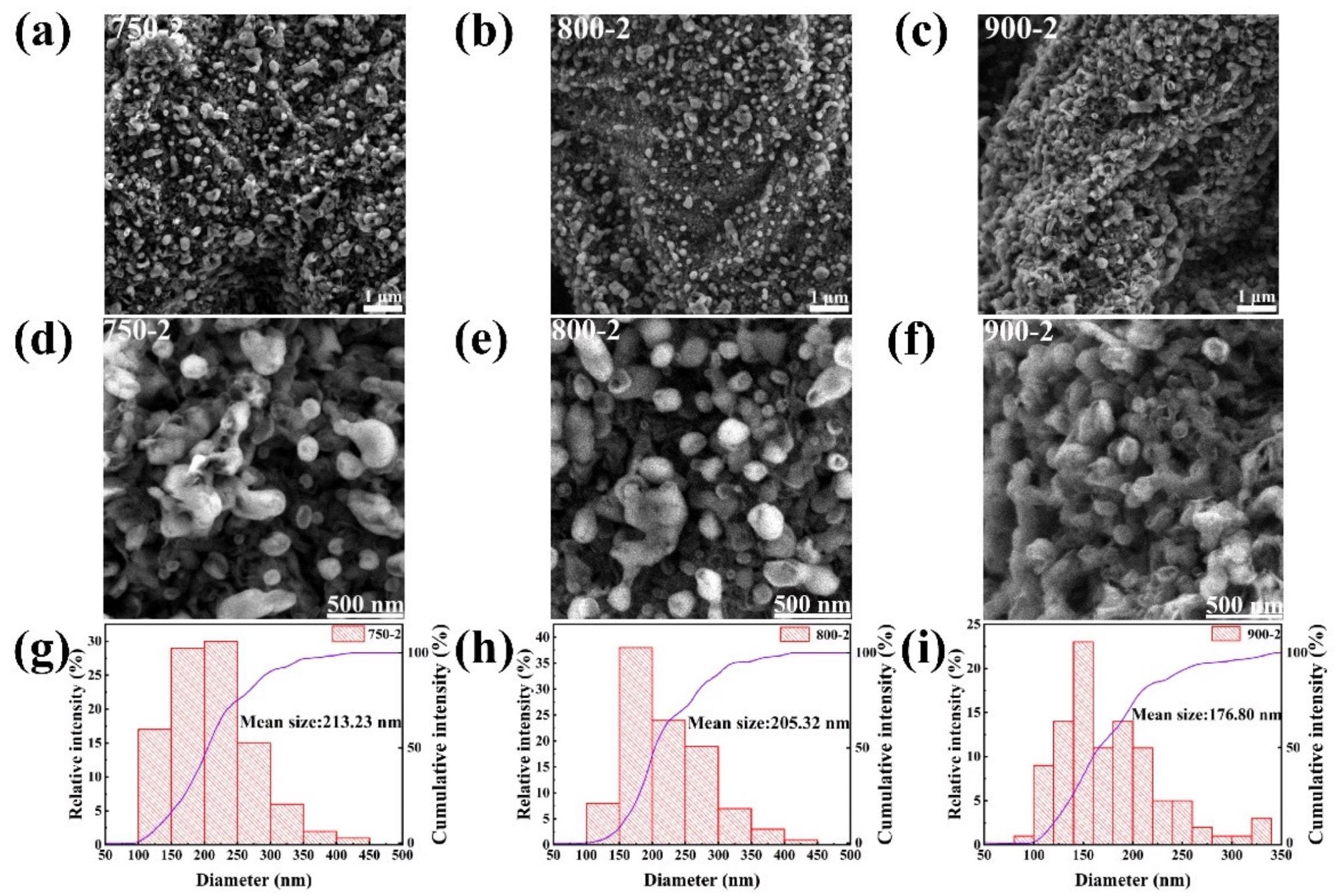
Figure 4. (a,d) SEM images of 750-2; (b,e) SEM images of 800-2; (c,f) SEM images of 900-2; (g–i) Particle size distribution of 750-2, 800-2, 900-2. Image Credit: Zeng, et al., 2022
The sp2 to sp3 ratios of samples 750-1, 800-1, and 900-1 are 0.99, 1.06, and 1.09, respectively, as illustrated in Figure 5a–c.
The sp2 to sp3 ratios of samples 750-2, 800-2, and 900-2 were 1.17, 1.39, and 1.51, respectively, as shown in Figures 5d and e. It can be seen that the degree of graphitization is closely proportional to temperature, regardless of whether procedure one or method two is utilized.
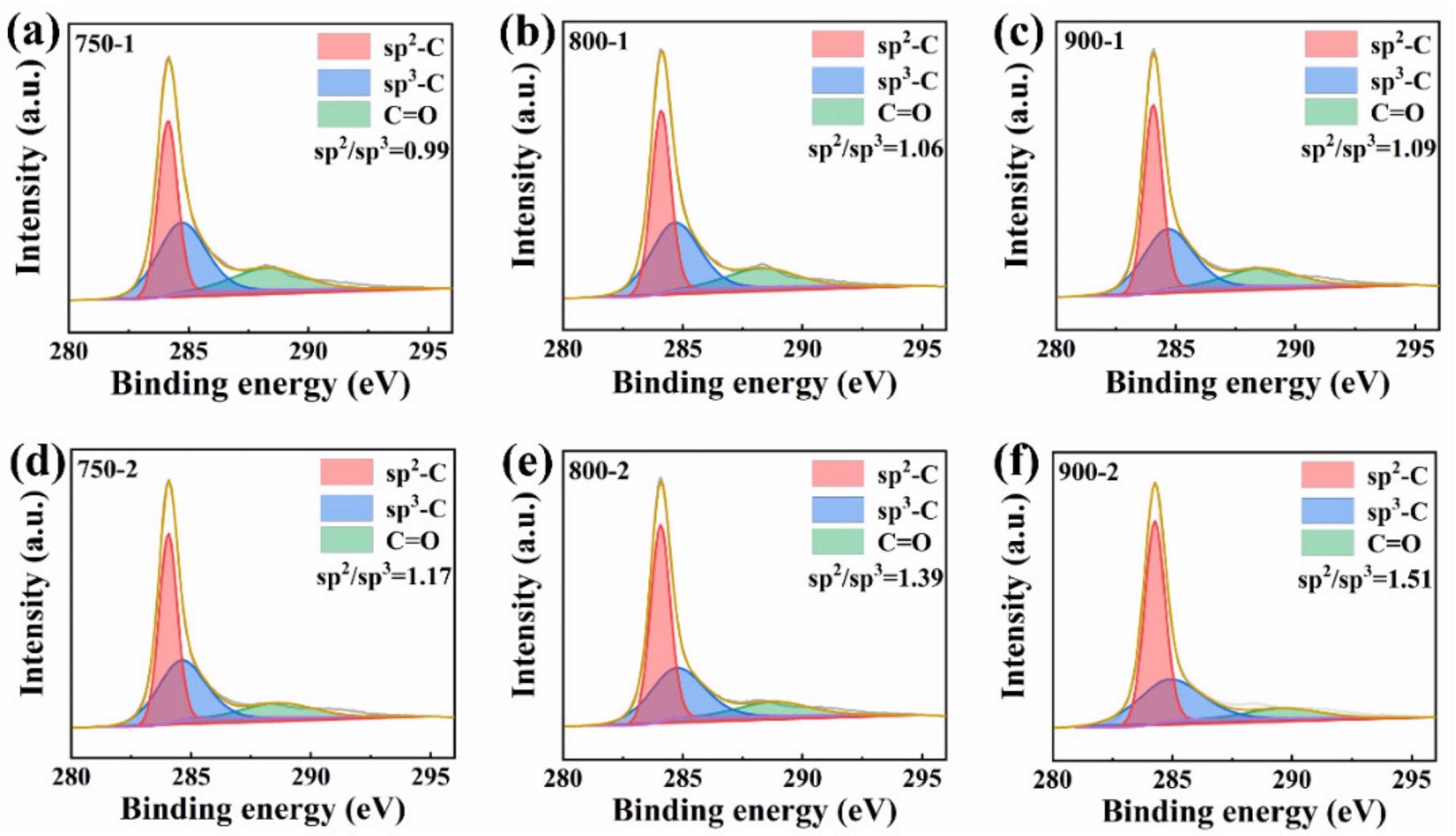
Figure 5. (a) XPS spectrum of C1s for 750-1; (b) XPS spectrum of C1s for 800-1; (c) C1s spectrum for 900-1; (d) C1s for 750-2; (e) XPS spectrum of C1s for 800-2; (f) XPS spectrum of C1s for 900-2. Image Credit: Zeng, et al., 2022
To learn more about method two, TEM combined with selected area electron diffraction (SAED) used to examine the structure and graphitization of 750-2, 800-2, and 900-2 samples (Figure 6). Figure 6a–c shows the SAED diffraction rings in great detail.
HRTEM images of 750-2, 800-2, and 900-2 are shown in Figure 6d, e. The lattice fringes grow more visible as the temperature rises, as can be seen in the graph.
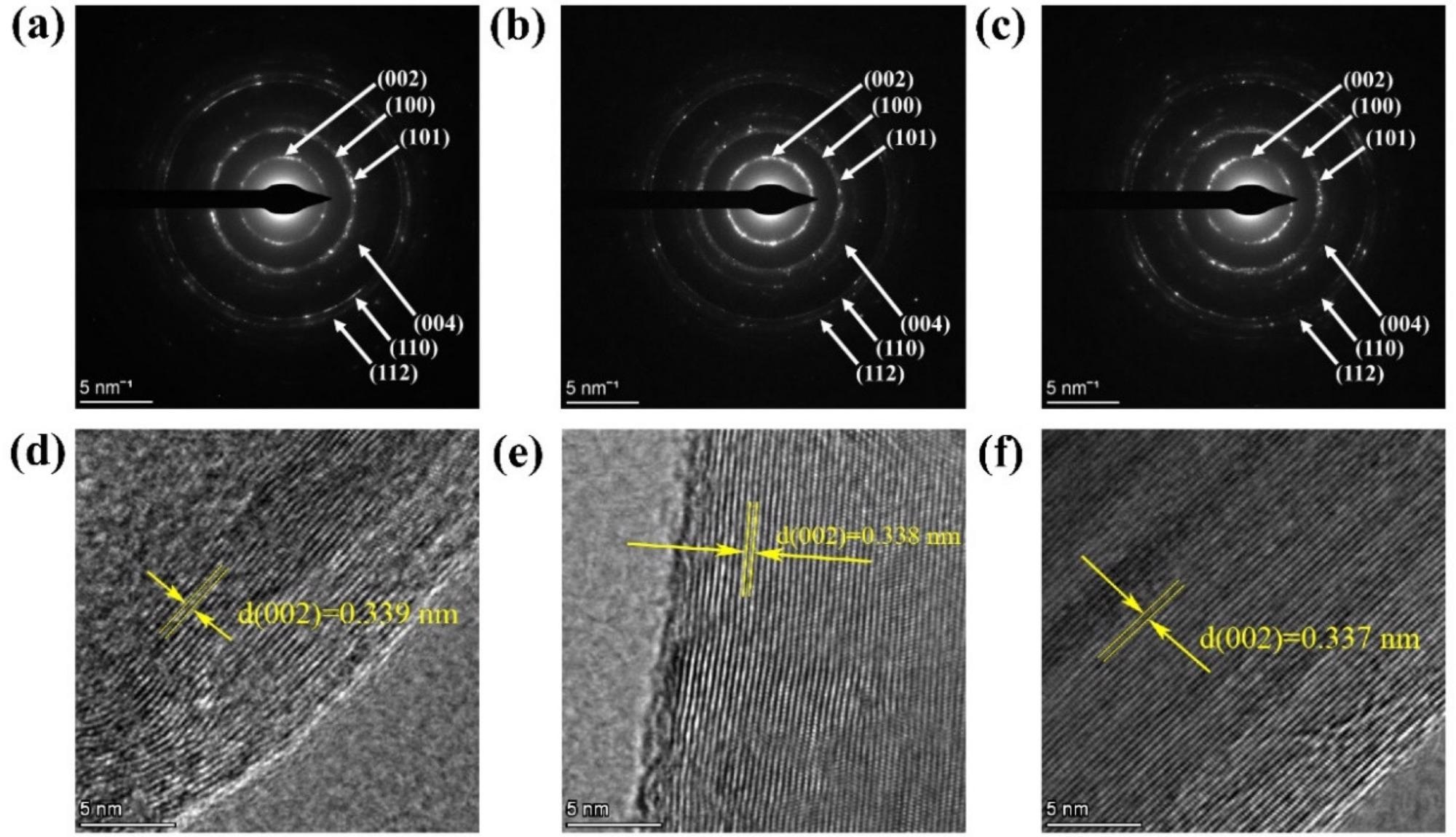
Figure 6. (a–c) SAED pattern of 750-2, 800-2, 900-2; (d–f) HRTEM images of 750-2, 800-2, 900-2. Image Credit: Zeng, et al., 2022
When the results of XRD, XPS, Raman, TEM, and SAED are combined, it is clear that graphitic carbon is formed by Ni catalysis.
Conclusion
Researchers refined the procedure for producing low-temperature, highly graphitized pomelo peel in this study, turning trash into a valuable material.
This study compares and contrasts improved and classic graphitization approaches. The degree of graphitization achieved with the new approach is 80.23% at 900 °C, which is somewhat lower than commercial graphite (90.23%) but significantly greater than the sample at 900 °C (48.8%) with the previous method.
At 900 °C, pomelo peels can be turned into highly graphitized nanospheres using the enhanced approach. The temperature plays a crucial role in the catalysis of pomelo peel by Ni, regardless of whether the classic approach or the enhanced method is used.
This research has the potential to transform pomelo peels into useful commodities, minimize pollution, and, most importantly, reduce power consumption and support sustainable development.
Journal Reference:
Zeng, L., Wang, Y., Guo, Y., Dai, X., Chen, L., He, C., Nhung, N. T. H., Wei, Y., Dodbiba, G., Fujita, T. (2022) Improved Method for Preparing Nanospheres from Pomelo Peel to Achieve High Graphitization at a Low Temperature. Crystals, 12(3), p. 403. Available Online: https://www.mdpi.com/2073-4352/12/3/403/htm.
References and Further Reading
- Xiao, L., et al. (2021) Utilization of pomelo peels to manufacture value-added products: A review. Food Chemistry, 351, p. 129247. doi.org/10.1016/j.foodchem.2021.129247.
- Chen, X., et al. (2020) Carbon footprint of a typical pomelo production region in China based on farm survey data. Journal of Cleaner Production, 277, p. 124041. doi.org/10.1016/j.jclepro.2020.124041.
- Cheng, D., et al. (2020) Feasibility study on a new pomelo peel derived biochar for tetracycline antibiotics removal in swine wastewater. Science of The Total Environment, 720, p. 137662. doi.org/10.1016/j.scitotenv.2020.137662.
- Wang, W & Chen, M (2022) Catalytic degradation of sulfamethoxazole by peroxymonosulfate activation system composed of nitrogen-doped biochar from pomelo peel: Important roles of defects and nitrogen, and detoxification of intermediates. Journal of Colloid and Interface Science, 613, pp. 57–70. doi.org/10.1016/j.jcis.2022.01.006.
- Wu, M. C., et al. (2019) Mesoporous carbon derived from pomelo peel as a high-performance electrode material for zinc-bromine flow batteries. Journal of Power Sources, 442, p. 227255. doi.org/10.1016/j.jpowsour.2019.227255.
- Chen, Q., et al. (2020) Biomass-derived porous graphitic carbon materials for energy and environmental applications. Journal of Materials Chemistry A, 8(12), pp. 5773–5811. doi.org/10.1039/C9TA11618D.
- Aval, L. F., et al. (2018) High-performance supercapacitors based on the carbon nanotubes, graphene and graphite nanoparticles electrodes. Heliyon Dentistry, 4(11), p. e00862. doi.org/10.1016/j.heliyon.2018.e00862.
- Ma, Y., et al. (2005) A practical route to the production of carbon nanocages. Carbon, 43(8), pp. 1667–1672. doi.org/10.1016/j.carbon.2005.02.004.
- Cassell, A. M., et al. (1999) Large scale CVD synthesis of single-walled carbon nanotubes. The Journal of Physical Chemistry B, 103(31), pp. 6484–6492. doi.org/10.1021/jp990957s.
- Sevilla, M., et al. (2008) Direct synthesis of graphitic carbon nanostructures from saccharides and their use as electrocatalytic supports. Carbon, 46(6), pp. 931–939. doi.org/10.1016/j.carbon.2008.02.019.
- Fan, C. L., et al. (2012) Structural developments of artificial graphite scraps in further graphitization and its relationships with discharge capacity. Electrochimica Acta, 75, pp. 311–315. doi.org/10.1016/j.electacta.2012.05.010.
- Huang, S., et al. (2013) Carbonization and graphitization of pitch applied for anode materials of high power lithium ion batteries. Journal of Solid State Electrochemistry, 17, pp. 1401–1408. doi.org/10.1007/s10008-013-2003-9.
- Zhang, S., et al. (2020) Structural order evaluation and structural evolution of coal derived natural graphite during graphitization. Carbon, 157, pp. 714–723. doi.org/10.1016/j.carbon.2019.10.104.
- Liu, H., et al. (2021) Sustainable utilization of wetland biomass for activated carbon production: A review on recent advances in modification and activation methods. Science of The Total Environment, 790, p. 148214. doi.org/10.1016/j.scitotenv.2021.148214.
- Danish, M & Ahmad, T (2018) A review on utilization of wood biomass as a sustainable precursor for activated carbon production and application. Renewable and Sustainable Energy Reviews, 87, pp. 1–21. doi.org/10.1016/j.rser.2018.02.003.
- Li, H., et al. (2020) Catalytic graphitization of coke carbon by iron: Understanding the evolution of carbon Structure, morphology and lattice fringes. Fuel, 279, p. 118531. doi.org/10.1016/j.fuel.2020.118531.
- Wang, K., et al. (2016) Nickel catalytic graphitized porous carbon as electrode material for high performance supercapacitors. Energy, 101, pp. 9–15. doi.org/10.1016/j.energy.2016.01.059.
- Thompson, E., et al. (2015) Iron-catalyzed graphitization of biomass. Green Chemistry, 17(1), pp. 551–556. doi.org/10.1039/C4GC01673D.
- Gomez-Martin, A., et al. (2018) Iron-Catalyzed Graphitic Carbon Materials from Biomass Resources as Anodes for Lithium-Ion Batteries. ChemSusChem, 11(16), pp. 2776–2787. doi.org/10.1002/cssc.201800831.
- Sevilla, M & Fuertes, A B (2010) Graphitic carbon nanostructures from cellulose. Chemical Physics Letters, 490(1–3), pp. 63–68. doi.org/10.1016/j.cplett.2010.03.011.
- Yan, Q., et al. (2018) Catalytic graphitization of kraft lignin to graphene-based structures with four different transitional metals. Journal of Nanoparticle Research, 20, p. 223. doi.org/10.1007/s11051-018-4317-0.
- Destyorini, F., et al. (2021) Temperature driven structural transition in the nickel-based catalytic graphitization of coconut coir. Diamond and Related Materials, 117, p. 108443. doi.org/10.1016/j.diamond.2021.108443.
- Fujimoto, H (2003) Theoretical X-ray scattering intensity of carbons with turbostratic stacking and AB stacking structures. Carbon, 41, pp. 1585–1592. doi.org/10.1016/S0008-6223(03)00116-7.
- Zou, L., et al. (2003) An investigation of heterogeneity of the degree of graphitization in carbon–carbon composites. Materials Chemistry and Physics, 82(3), pp. 654–662. doi.org/10.1016/S0254-0584(03)00332-8.
- Qiu, T., et al. (2019) The preparation of synthetic graphite materials with hierarchical pores from lignite by one-step impregnation and their characterization as dye absorbents. RSC Advances, 9(22), pp. 12737–12746. doi.org/10.1039/C9RA00343F.
- Sevilla, M., et al. (2007) Synthesis of graphitic carbon nanostructures from sawdust and their application as electrocatalyst supports. The Journal of Physical Chemistry C, 111(27), pp. 9749–9756. doi.org/10.1021/jp072246x.
- Destyorini, F., et al. (2021) Formation of nanostructured graphitic carbon from coconut waste via low-temperature catalytic graphitisation. Engineering Science and Technology, an International Journal, 24(2), pp. 514–523. doi.org/10.1016/j.jestch.2020.06.011.
- Zha, Z., et al. (2021) Porous graphitic carbon from mangosteen peel as efficient electrocatalyst in microbial fuel cells. Science of The Total Environment, 764, p. 142918. doi.org/10.1016/j.scitotenv.2020.142918.
- Hu, C., et al. (2015) Raman spectroscopy study of the transformation of the carbonaceous skeleton of a polymer-based nanoporous carbon along the thermal annealing pathway. Carbon, 85, pp. 147–158. doi.org/10.1016/j.carbon.2014.12.098.
- Kim, T., et al. (2016) Full graphitization of amorphous carbon by microwave heating. RSC Advances, 6(29), pp. 24667–24674. doi.org/10.1039/C6RA01989G.
- Liu, X., et al. (2015) Biomass-derived nitrogen self-doped porous carbon as effective metal-free catalysts for oxygen reduction reaction. Nanoscale, 7(14), pp. 6136–6142. doi.org/10.1039/C5NR00013K.
- Schuepfer, D. B., et al. (2020) Assessing the structural properties of graphitic and non-graphitic carbons by Raman spectroscopy. Carbon, 161, pp. 359–372. doi.org/10.1016/j.carbon.2019.12.094.
- Chu, P K & Li, L (2006) Characterization of amorphous and nanocrystalline carbon films. Materials Chemistry and Physics, 96(2–3), pp. 253–277. doi.org/10.1016/j.matchemphys.2005.07.048.
- Bao, C., et al. (2021) Purification effect of the methods used for the preparation of the ultra-high purity graphite. Diamond and Related Materials, 120, p. 108704. doi.org/10.1016/j.diamond.2021.108704.
- Gong, Y., et al. (2017) Highly porous graphitic biomass carbon as advanced electrode materials for supercapacitors. Green Chemistry, 19(17), pp. 4132–4140. doi.org/10.1039/C7GC01681F.
- Huang, H., et al. (2021) Efficient activation of persulfate by a magnetic recyclable rape straw biochar catalyst for the degradation of tetracycline hydrochloride in water. Science of The Total Environment, 758, p. 143957. doi.org/10.1016/j.scitotenv.2020.143957.
- Liou, T. H., et al. (2022) Sustainable utilization of rice husk waste for preparation of ordered nanostructured mesoporous silica and mesoporous carbon: Characterization and adsorption performance. Colloids and Surfaces A: Physicochemical and Engineering Aspects, 636, p. 128150. doi.org/10.1016/j.colsurfa.2021.128150.
- Hou, K., et al. (2018) Composition, characteristics and antioxidant activities of fruit oils from Idesia polycarpa using homogenate-circulating ultrasound-assisted aqueous enzymatic extraction. Industrial Crops and Products, 117, pp. 205–215. doi.org/10.1016/j.indcrop.2018.03.001.
- Cao, L., et al. (2022) Straw and wood based biochar for CO2 capture: Adsorption performance and governing mechanisms. Separation and Purification Technology, 287, p. 120592. doi.org/10.1016/j.seppur.2022.120592.
- Anton, R (2008) On the reaction kinetics of Ni with amorphous carbon Carbon, 46, pp. 656–662. doi.org/10.1016/j.carbon.2008.01.021.
- Zhang, P., et al. (2019) Catalytic degradation of estrogen by persulfate activated with iron-doped graphitic biochar: Process variables effects and matrix effects. Chemical Engineering Journal, 378, p. 122141. doi.org/10.1016/j.cej.2019.122141.
- Gai, L., et al. (2021) Evolution of biomass to porous graphite carbon by catalytic graphitization. Journal of Environmental Chemical Engineering, 9(6), p. 106678. doi.org/10.1016/j.jece.2021.106678.