The complete and precise identification of diseases, as well as the monitoring of physiologically relevant compounds in body fluids, is a major component of modern medicine.
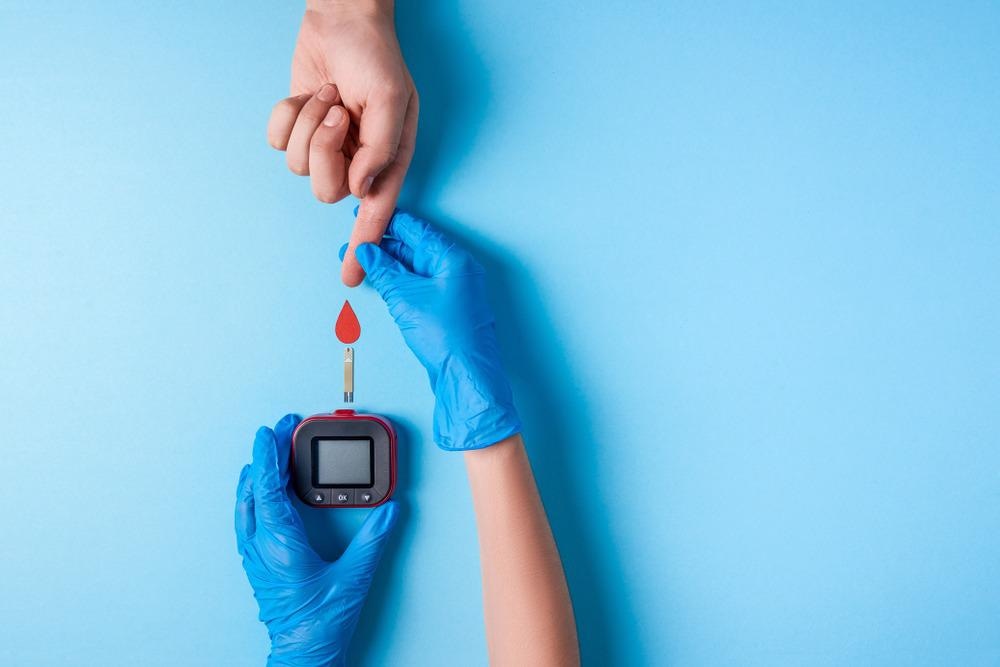
Image Credit: Kateryna Novikova/Shutterstock.com
Diabetes mellitus, sometimes known as diabetes, is a chronic metabolic condition that has become one of the world’s most serious health issues. The fasting glucose level in the blood of a healthy person varies from 3.9–5.6 mM, but diabetes patients’ glucose levels are higher.
The glucose content in biological fluids is determined using various analytical techniques, mostly optical and electrochemical. Because of their simplicity, high sensitivity and selectivity, suitable linear range, and low limit of detection (LOD), electrochemical enzymatic biosensors based on glucose oxidase (GOx) are the most extensively utilized among these approaches.
However, biosensor performance can be increased by depositing nanostructures on the electrode surface by selecting the most appropriate GOx immobilization technique.
Direct deposition of gold nanostructures (GNs) on the surface of the electrode utilizing constant potential amperometry (CPA), differential pulse voltammetry, pulse amperometry, and square-wave voltammetry is a simple and effective method for ensuring the formation of nanostructures of various shapes and sizes.
GNs-modified electrodes have a large surface area, which allows for higher enzyme loading, better orientation, and more efficient electrical contact during electrochemical processes, good chemical stability and resistance to surface poisoning during electrochemical processes, catalytic efficiency, high conductivity, and biocompatibility.
GNs-modified electrodes boost the electrocatalytic activity of enzymes, offer direct electron transfer in some cases, and improve the performance of biofuel cells due to these features.
Due to its superhydrophobicity, distinct size, and shape-dependent features, such as improved SERS sensitivity, electrocatalytic activity, and photoluminescence emission, dendritic gold nanostructures (DGNs) have been a huge success among diverse shaped GNs.
Adsorption is the quickest and most straightforward way, but the enzyme layer is unstable, and the electrodes are inconsistent. The GOx is covalently immobilized on a self-assembled monolayer (SAM), allowing for spatial control of the enzyme.
GA (glutaraldehyde) is a well-known cross-linker that contains reactive aldehyde groups. It can be utilized as a cross-linker via amino groups following enzyme adsorption on the electrode surface or for surface activation and covalent enzyme immobilization on various surfaces.
The goal of this research was to examine several GOx immobilization techniques on DGNs to produce a stable, selective, and sensitive amperometric glucose biosensor that works well in actual samples. The CPA approach was used to optimize the electrochemical deposition of DGNs on the electrode surface.
The performance of the developed glucose biosensors was characterized using three GOx immobilization methods: simple adsorption on DGNs and cross-linking with GA vapor (first method), covalent immobilization on DGNs modified with 11-mercaptoundecanoic acid SAM (second method), and covalent immobilization on SAM with additional cross-linking with GA vapor (third method).
Methodology
The electrochemical deposition of DGNs and electrochemical measurements were carried out with a programmed potentiostat/galvanostat Autolab PGSTAT30 (EcoChemie, The Netherlands), which was controlled by the NOVA1.9 software.
A cyclic voltammogram in 0.5 M H2SO4 electrolyte solution was used to evaluate the electroactive surface area of electrodeposited DGNs. The electroactive surface area of gold was calculated using the integrated peak of gold oxide reduction and a conversion factor of 386 µC cm-2.
All experiments were carried out in a 5 mL capacity electrochemical cell stirring the solution with a magnetic stirrer at +20 ± 2 °C (120 rpm). All electrochemical readings are provided as the average of three independent experiments.
The correlation coefficient (R) and determination coefficient (R2) of the calibration curve were investigated. The LOD was estimated as the lowest concentration of analytics that produces an analytical signal larger than the background value plus 3σ times the background value.
The electrochemical cell’s artificial redox mediator PMS is engaged in the reoxidation of the GOx redox center, and the resultant PMSH2 (the reduced form of PMS) is reoxidized on the working electrode’s surface (Scheme 1).
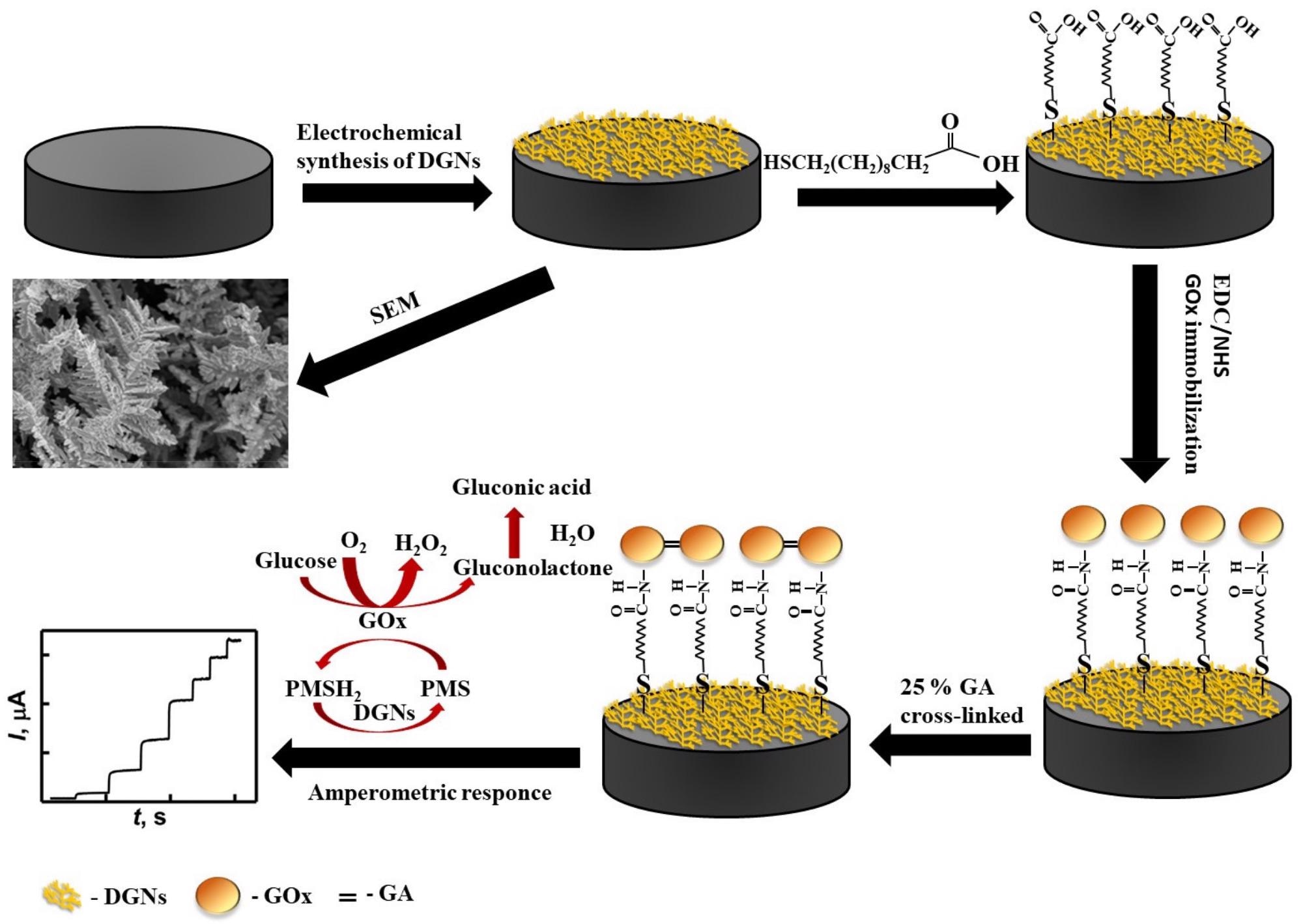
Scheme 1. Schematic representation of the modification of the GR electrode by DGNs, immobilization of GOx using the third method, and electrochemical determination of glucose by the developed biosensor. Credit: Sakalauskiene, et al., 2022
Results
DGNs were effectively electrochemically deposited on the GR electrode in both circumstances, according to FE-SEM images (Figure 1A, B). Longer, more orderly DGNs emerged after 400 seconds of synthesis (Figure 1B, B1), whereas shorter, sharper, and more branching DGNs emerged after 200 seconds (Figure 1A, A1). Figure 1C shows the predominance of Au and C elements in the EDS spectra of DGNs electrodeposited for 200 seconds on the GR electrode.
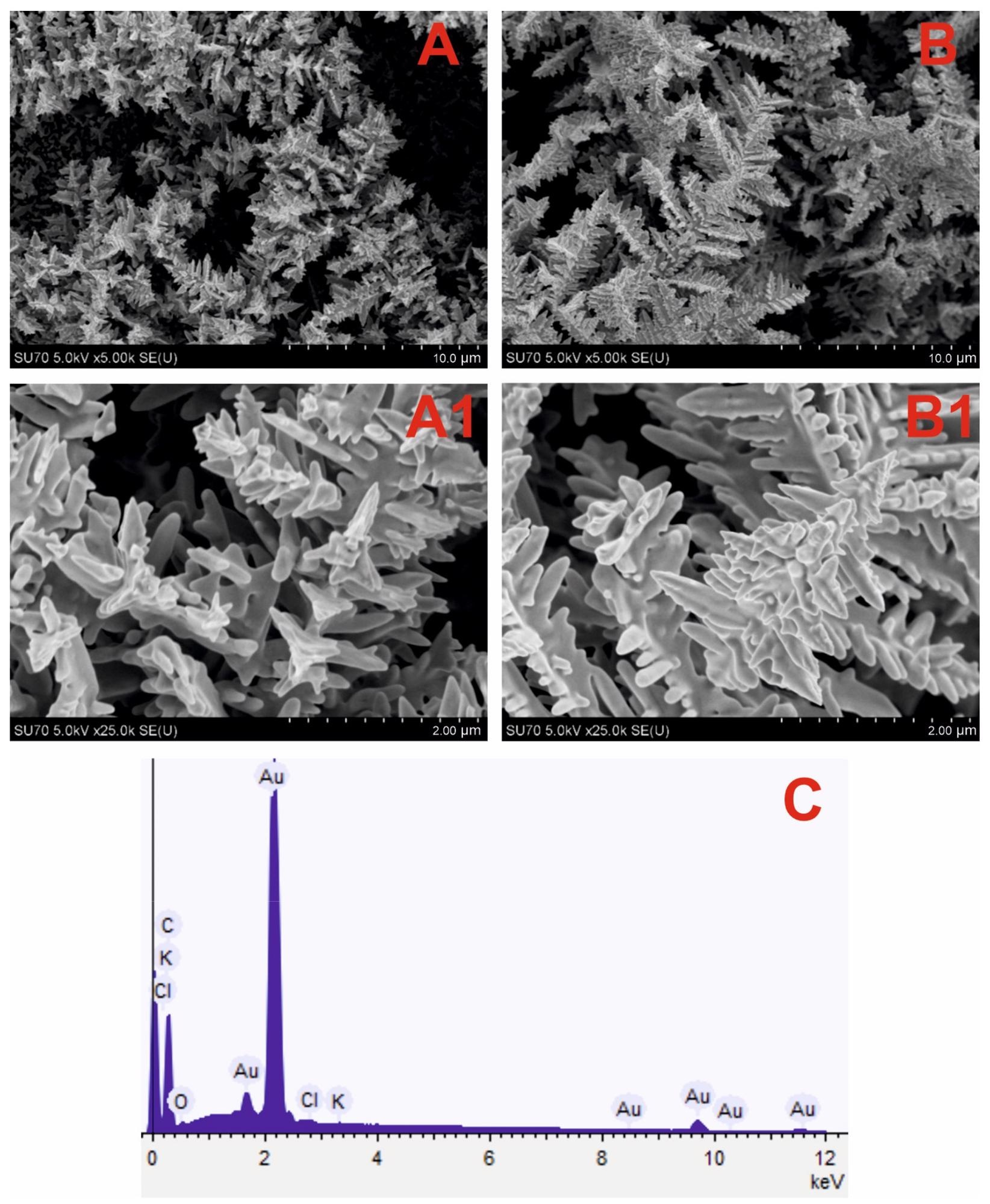
Figure 1. SEM images of the DGNs electrochemically deposited on a GR electrode at a constant −0.2 V potential for 200 seconds (A, A1) and 400 seconds (B, B1) from a 10 mM solution of HAuCl4 containing 0.1 M KNO3 (pictures (A1, B1) are magnified images of (A, B)) and EDS spectrum (C) of dendritic gold nanostructures presented in (A) SEM image. Image Credit: Sakalauskiene, et al., 2022
The amperometric signals observed at glucose concentrations of 5, 25, and 50 mM for both DGNs electrodeposition periods were clearly identical (Figure 2).
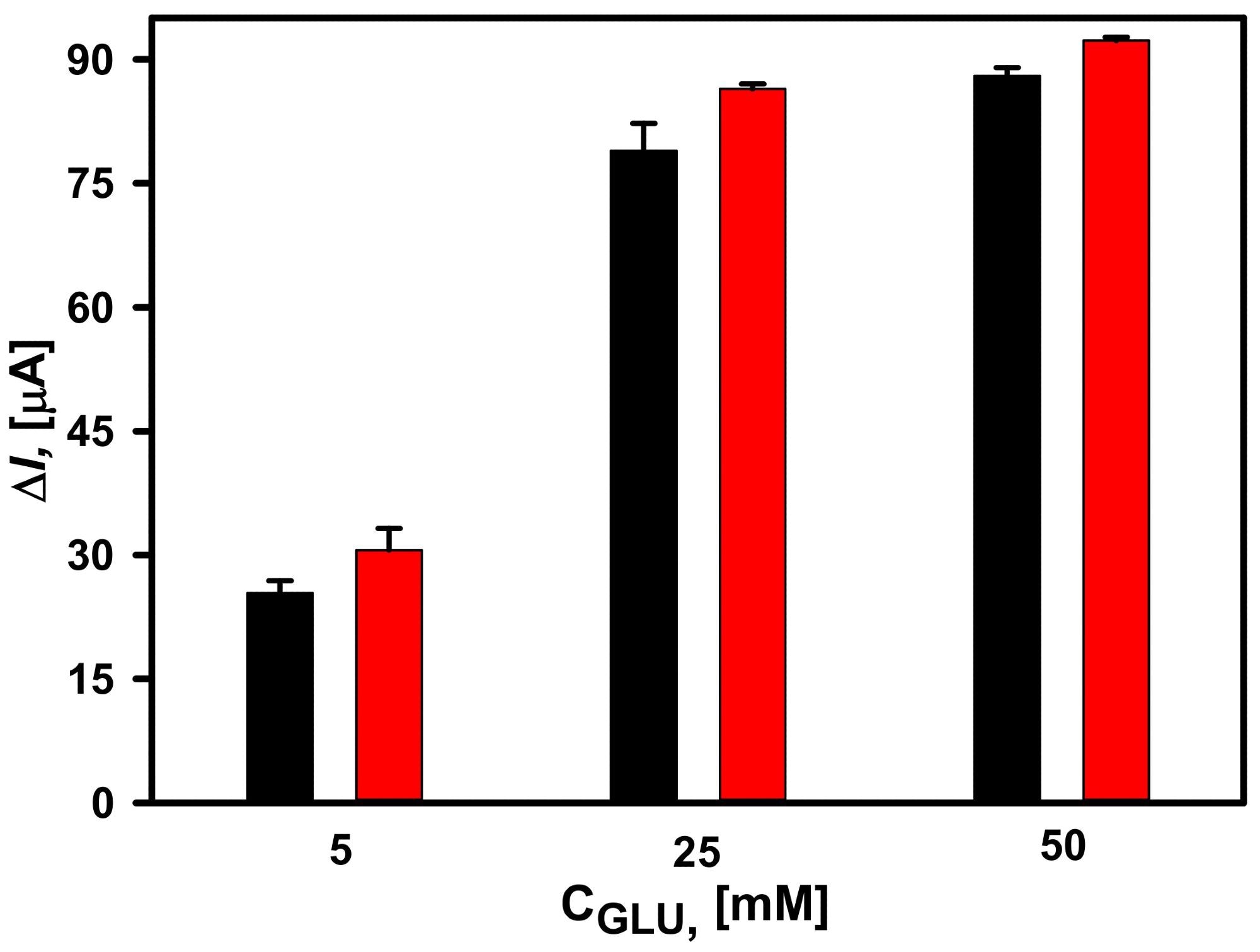
Figure 2. Dependence of the current response of glucose biosensors based on electrochemically deposited DGNs from a 10 mM HAuCl4 solution with 0.1 M KNO3 at a constant −0.2 V potential for 200 seconds (red column) and 400 seconds (black column). The amperometric response at +0.3 V was registered in 0.05 M SA buffer with 0.1 M KCl (pH 6.0) and 2.0 mM PMS. Image Credit: Sakalauskiene, et al., 2022
In addition, the electroactive surface area of electrodeposited DGNs was calculated using cyclic voltammetry measurements (Figure 3) in 0.5 M H2SO4.
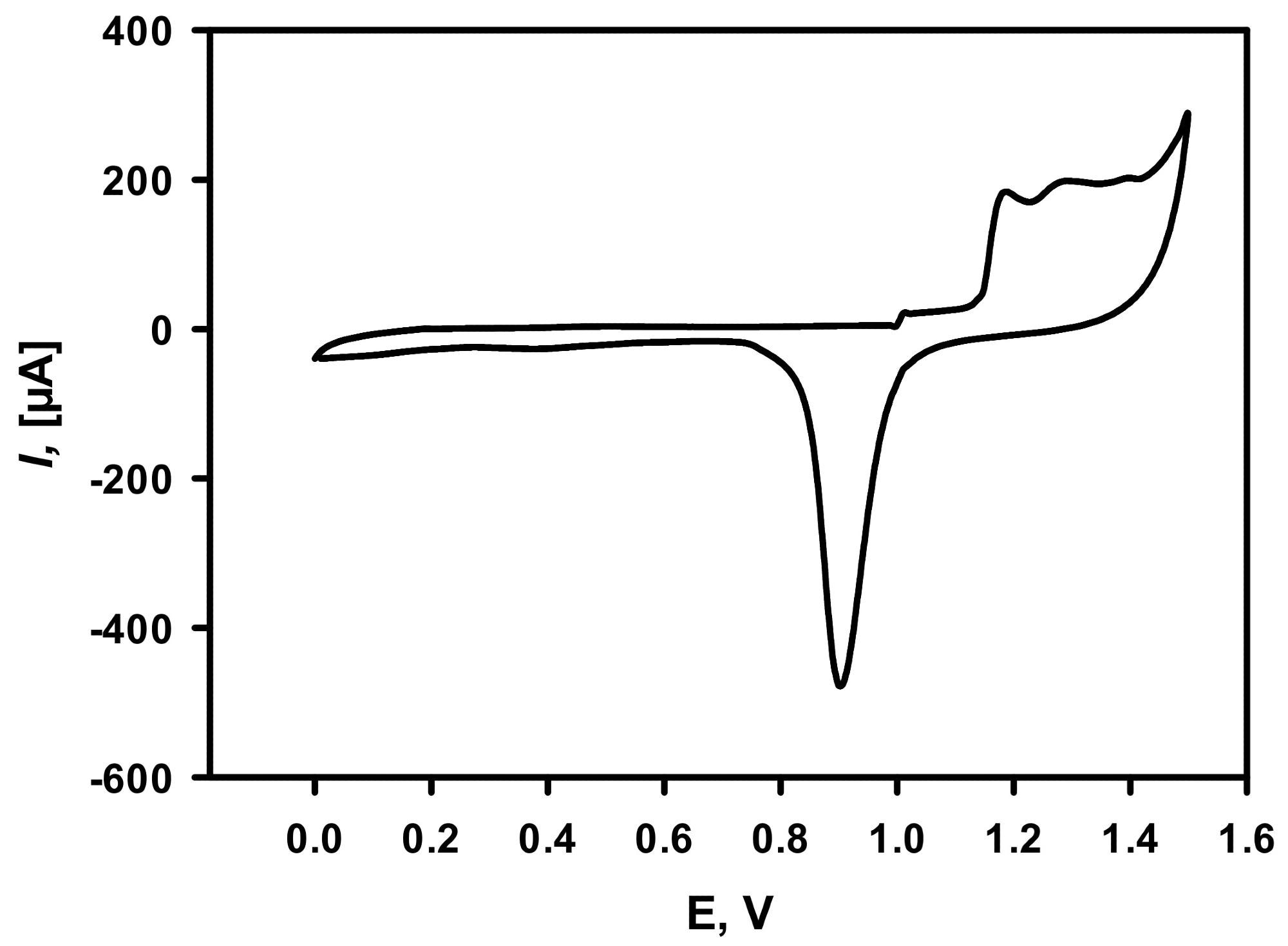
Figure 3. Cycling voltammogram of DGNs deposited on the GR electrode recorded in 0.5 M H2SO4. The scan rate was 0.1 V/s. DGNs electrodeposition was performed at a constant −0.2 V potential for 200 seconds from a 10 mM solution of HAuCl4 containing 0.1 M KNO3. Image Credit: Sakalauskiene, et al., 2022
All calibration curves had a hyperbolic connection between recorded currents and glucose concentrations in the range of 0.1 to 100 mM. (Figure 4A). The glucose biosensor depending on the GA-GOx-SAM/DGNs/GR electrode had a 1.41 times higher ΔImax than the GA-GOx/DGNs/GR electrode, but all three GOx immobilization techniques had the same linear dynamic range (LDR) from 0.1 to 10 mM. (Figure 4B).
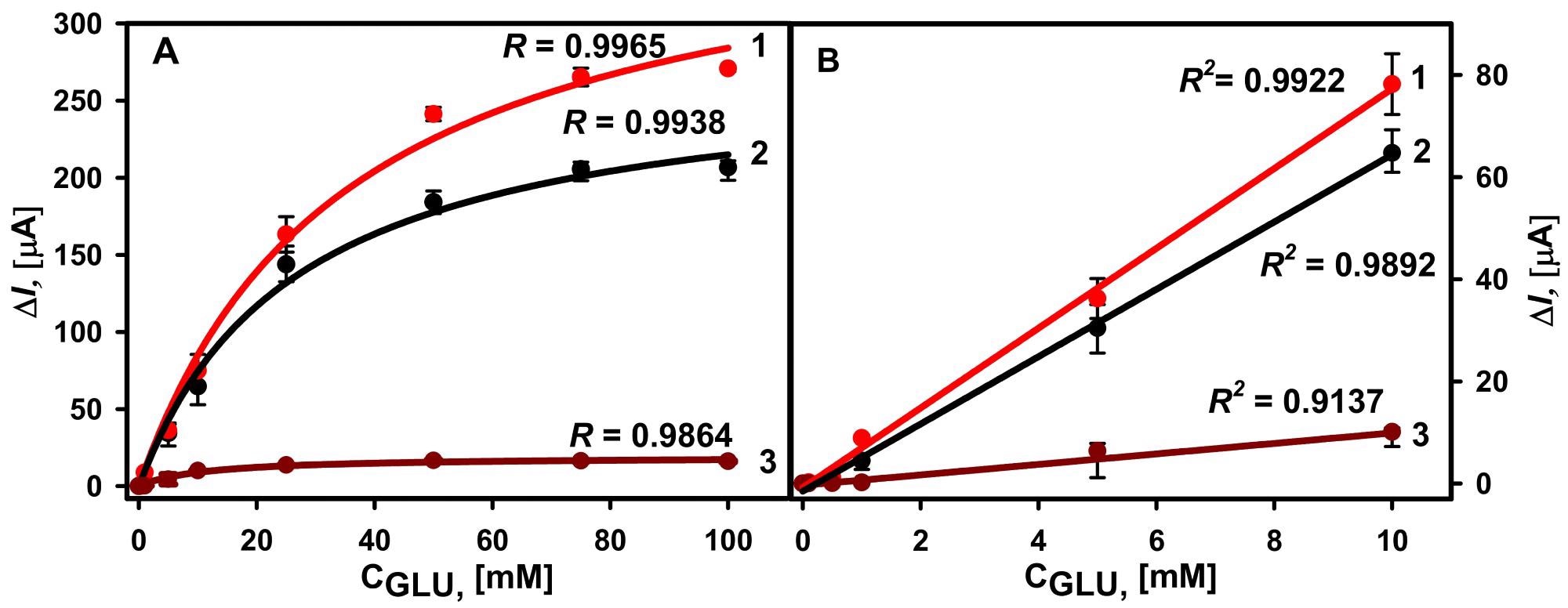
Figure 4. (A) Calibration plots of glucose biosensors based on GA-GOx-SAM/DGNs/GR (curve 1, third GOx immobilization method, red dots ●), GA-GOx/DGNs/GR (curve 2, first GOx immobilization method, black dots ●), and GOx-SAM/DGNs/GR electrodes (curve 3, second GOx immobilization method, brown dots ●). (B) The linear dynamic ranges of the developed biosensors. The amperometric response at +0.3 V was registered in 0.05 M SA buffer with 0.1 M KCl (pH 6.0) in the presence of 6.0 mM PMS. Image Credit: Sakalauskiene, et al., 2022
Table 1 shows a comparison of GOx immobilization techniques on the performance of second-generation glucose biosensors based on the use of various gold nanostructures.
Table 1. Comparison of glucose oxidase immobilization method on the performance of glucose biosensors with different gold nanostructures. Source: Sakalauskiene, et al., 2022
GOx Immobilization Method |
LOD/Sensitivity |
LDR |
Method/Mediator |
Ref. |
GOx adsorbed on the dendritic gold nanostructures modified graphite rod electrode and cross-linked with glutaraldehide vapour |
0.059 mM |
0.1–9.97 mM |
Amperometry
Phenazine methosulfate |
[11] |
GOx and 2,5-dihydroxybenzaldehyde cross-linked with glutaraldehyde and adsorbed on nanocomposite consisting of gold nanoparticles/reduced graphene oxide within gelatin deposited on a screen-printed electrode |
0.640 mM |
1–11 mM |
Cyclic voltammetry
2,5-dihydroxybenzaldehyde |
[33] |
GOx adsorbed on gold nanoparticles (3.5 nm) and redox mediator modified graphite rod electrode and cross-linked with glutaraldehide vapour |
0.024 mM
52.1 μA mM−1 cm−2 |
0.1–10 mM |
Amperometry
1,10-phenanthroline-5,6-dione |
[12] |
GOx adsorbed on gold nanoparticles electrochemically deposited on graphite rod electrode and cross-linked with glutaraldehide vapour |
0.083 mM
101.02 μA mM−1 cm−2 |
0.1–10 mM |
Amperometry
Phenazine methosulfate |
[7] |
GOx covalent attachment to gold nanoparticles (2.6 nm) monolayer modified gold electrode |
0.0082 mmol L−1
8.8 μA mM−1 cm−2 |
0.02–5.7 mM |
Amperometry
Ferrocenemethanol |
[34] |
GOx covalently immobilised on nanoporous gold modified with self-assembled monolayers (3,3′-dithiodipropionic acid/6-mercaptohexanoic acid/11-mercaptoundecanoic acid) via carbodiimide chemistry |
2.187 μA mM−1 cm−2
1.564 μA mM−1 cm−2
1.160 μA mM−1 cm−2 |
0.1–10 mM |
Amperometry
p-benzoquinone |
[35] |
Multilayers of GOx covalently immobilised on the gold electrode and gold nanoparticles (12 nm) obtained using cysteamine (6 layers) |
0.008 mM
5.72 μA mM−1 cm−2 |
0.01–13 mM |
Amperometry
Ferrocenemethanol |
[36] |
GOx covalently immobilized on 11-MUA SAM modified DGNs/GR electrode and cross-linked with GA vapour (GA-GOx-SAM/DGNs/GR) |
0.019 mM |
0.1 to 10 mM |
Amperometry
Phenazine methosulfate |
This work |
GOx adsorbed on the DGNs/GR electrode and cross-linked with GA vapour (GA-GOx/DGNs/GR) |
0.022 mM |
0.1 to 10 mM |
Amperometry
Phenazine methosulfate |
This work |
Figure 5 shows that a biosensor based on the GA-GOx-SAM/DGNs/GR electrode has higher repeatability.
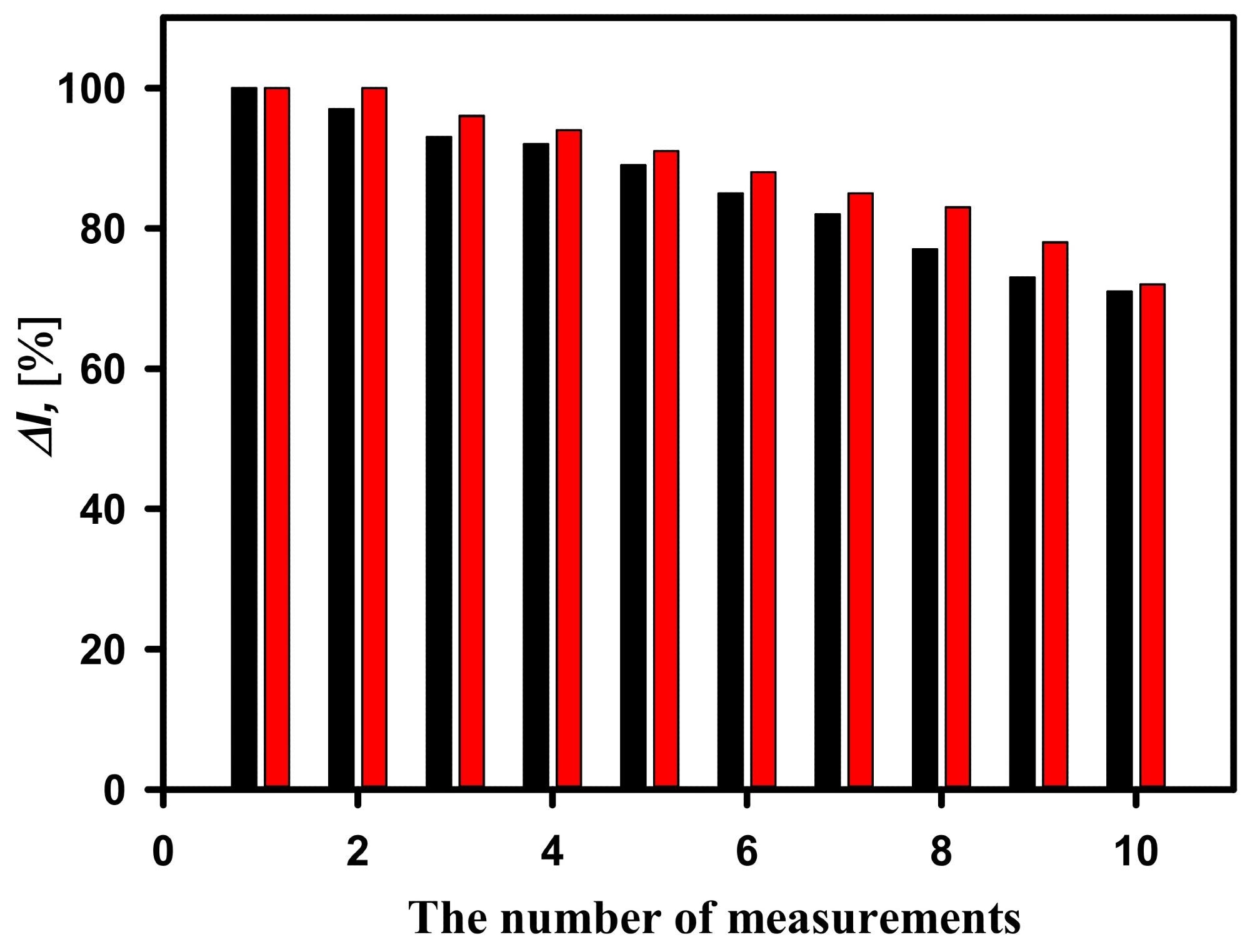
Figure 5. Current responses of the developed biosensors based on GA-GOx-SAM/DGNs/GR electrode (red columns) and GA-GOx/DGNs/GR electrodes (black columns) during repeated analytic detection. The current response was recorded at 25 mM of glucose concentration. Image Credit: Sakalauskiene, et al., 2022
For the two types of electrodes, hyperbolic relationships between recorded currents in diluted serum and added glucose concentration range of 0.1 to 100 mM were established (Figure 6A). Despite the variation in KM(app), the LDRs (Figure 6B) were the same and no changes were observed depending on the GOx immobilization approach.
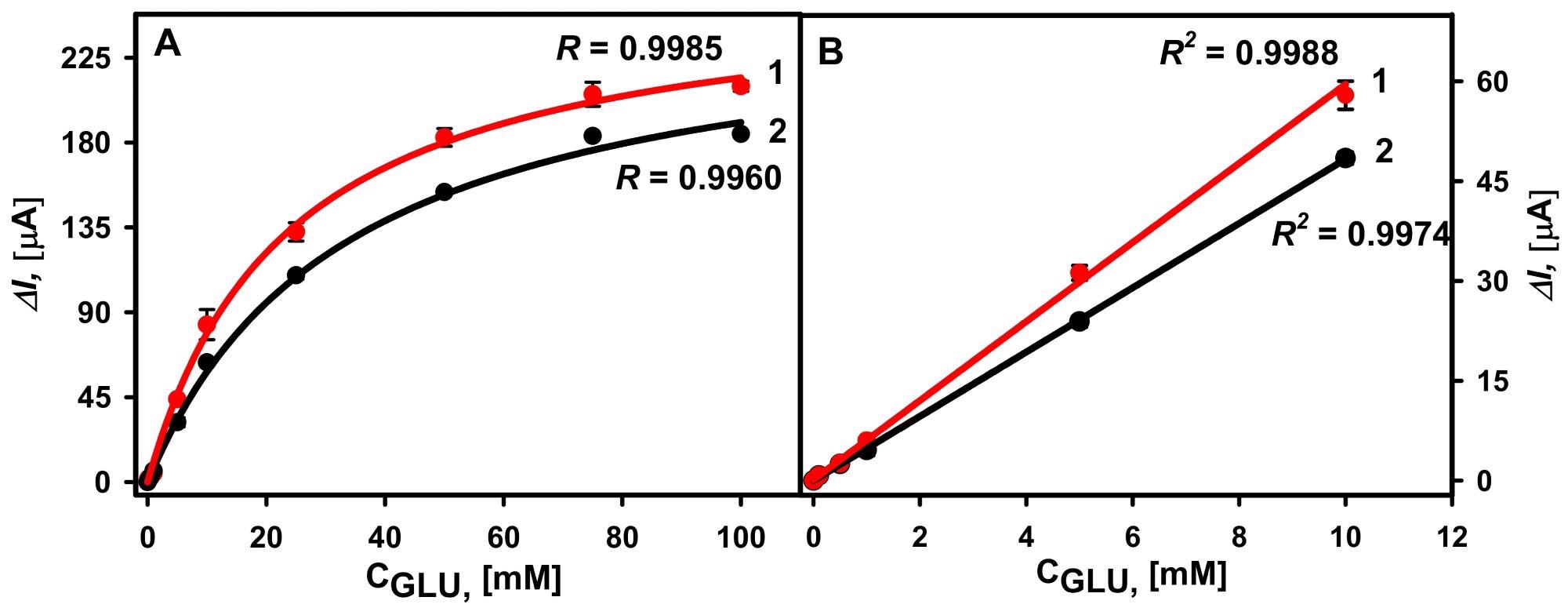
Figure 6. (A) Calibration plots of glucose biosensors based on GA-GOx-SAM/DGNs/GR (curve 1, red dots ●) and GA-GOx/DGNs/GR (curve 2, black dots ●) electrodes in serum. (B) The linear glucose detection range. The amperometric response at +0.3 V was registered in 0.05 M SA buffer with 0.1 M KCl (pH 6.0) diluted serum in the presence of 6.0 mM PMS. Image Credit: Sakalauskiene, et al., 2022
In addition, the impact of ascorbic and uric acids as probable interfering agents on glucose measurement was investigated (Figure 7).
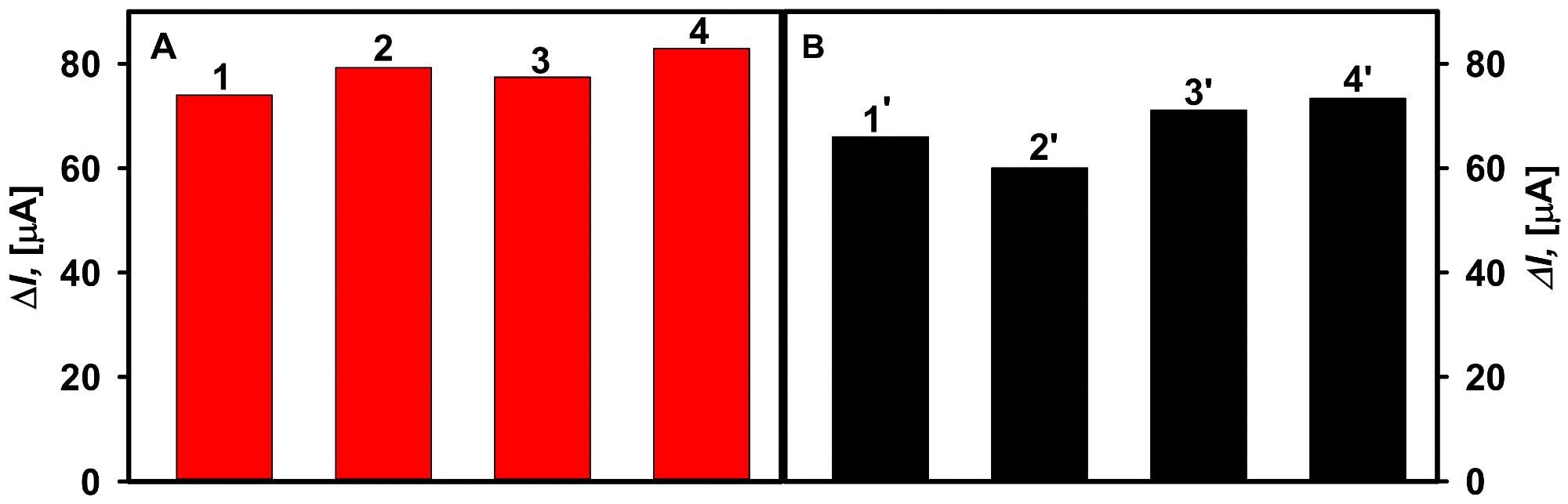
Figure 7. The effect of interfering substances on the current response of the glucose biosensors developed based on GA-GOx-SAM/DGNs/GR (A) and GA-GOx/DGNs/GR (B) electrodes. Diagrams of the registered current response are presented after addition of: 10 mM solution of glucose (column 1, 1′), 10 mM solution of glucose with 0.1 mM uric acid (column 2, 2′), 10 mM solution of glucose with 0.01 mM ascorbic acid (column 3, 3′), and 10 mM solution of glucose with 0.05 mM ascorbic acid (column 4, 4′). Image Credit: Sakalauskiene, et al., 2022
During a 32-day period, the reliability of glucose biosensors based on GA-GOx-SAM/DGNs/GR and GA-GOx/DGNs/GR electrodes was examined (Figure 8).
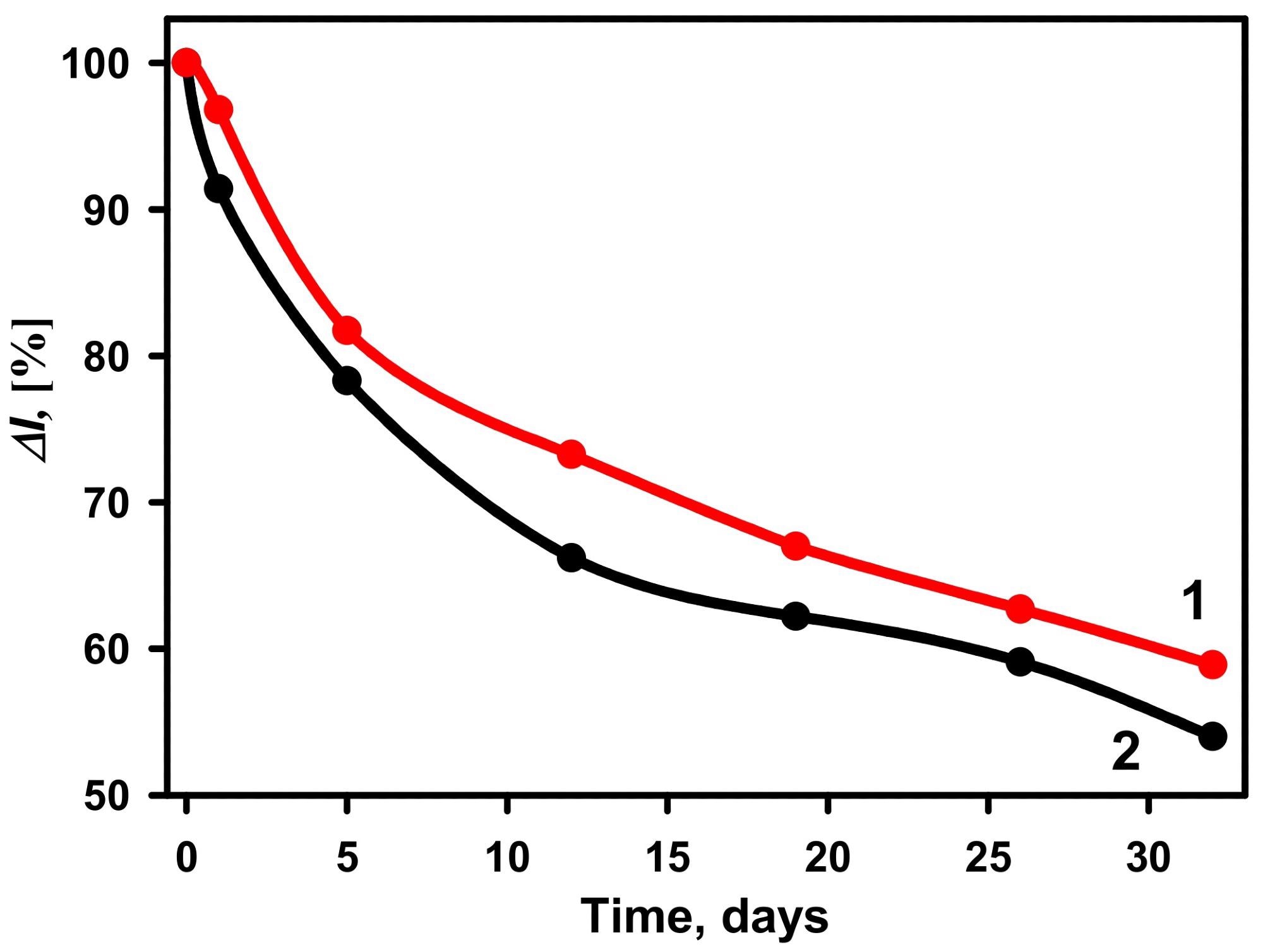
Figure 8. The stability of biosensors based on GA-GOx-SAM/DGNs/GR (curve 1, red dots ●) and GA-GOx/DGNs/GR (curve 2, black dots ●) electrodes. The amperometric response was measured in 0.05 M SA buffer with 0.1 M KCl (pH 6.0) and 6.0 mM PMS. Image Credit: Sakalauskiene, et al., 2022
Conclusion
The electrochemical deposition of DGNs on a GR electrode was optimized in this study at a constant voltage in time. Furthermore, it was discovered that the chosen GOx immobilization technique on DGNs has a significant impact on the performance of the created glucose biosensors in the buffer solution and serum.
The use of GA to cross-link GOx after covalent immobilization on the SAM increased the biosensing layer’s sensitivity and stability, as well as the repeatability of the current response after many glucose detections using the created biosensor.
Although this immobilization method reduces enzyme loss during continuous amperometric measurements in solution with mixing, DGNs are delicate. They can be destroyed or removed from the surface along with enzymes under unsuitable experimental circumstances.
Furthermore, covalently mounted multilayers of GOx on gold nanostructures are a potential avenue for increasing biosensor analytical parameters. To make the operation of such biosensors easier, a reagent-free system with the redox mediator immobilized on the surface of DGNs/GR electrodes must be developed.
Journal Reference:
Sakalauskiene, L., Popov, A., Kausaite-Minkstimiene, A., Ramanavicius, A. and Ramanaviciene, A. (2022) The Impact of Glucose Oxidase Immobilization on Dendritic Gold Nanostructures on the Performance of Glucose Biosensors. Biosensors, 12(5), p.320. Available Online: https://www.mdpi.com/2079-6374/12/5/320/htm.
References and Further Reading
- Diabetes (2022) Available online: https://www.who.int/news-room/fact-sheets/detail/diabetes.
- Mean Fasting Blood Glucose. Available online: https://www.who.int/data/gho/indicator-metadata-registry/imr-details/2380
- Yu, Z., et al. (2021) Optical sensors for continuous glucose monitoring. Progress in Biomedical Engineering, 3, p. 22004.
- Witkowska Nery, E., et al. (2016) Electrochemical Glucose Sensing: Is There Still Room for Improvement? Analytical Chemistry, 88, pp. 11271–11282. doi.org/10.1021/acs.analchem.6b03151.
- Donmez, S (2020) A novel electrochemical glucose biosensor based on a poly (L-aspartic acid)-modified carbon-paste electrode. Preparative Biochemistry & Biotechnology, 50, pp. 961–967. doi.org/10.1080/10826068.2020.1805758.
- Ullah, S., et al. (2018) In-vitro model for assessing glucose diffusion through skin. Biosensors and Bioelectronics, 11, pp. 175–179. doi.org/10.1016/j.bios.2018.03.039.
- German, N., et al. (2014) Electrochemical deposition of gold nanoparticles on graphite rod for glucose biosensing. Sensors & Actuators, B: Chemical, 203, pp. 25–34. doi.org/10.1016/j.snb.2014.06.021.
- House, J. L., et al. (2007) Immobilization Techniques to Avoid Enzyme Loss from Oxidase-Based Biosensors: A One-Year Study. Journal of Diabetes Science and Technology, 1, pp. 18–27. doi.org/10.1177%2F193229680700100104.
- Das, A. K., et al. (2015) Nicotinamide adenine dinucleotide assisted direct electrodeposition of gold nanodendrites and its electrochemical applications. Electrochimica Acta, 158, pp. 129–137. doi.org/10.1016/j.electacta.2015.01.119.
- Shu, H., et al. (2014) Direct Electrodeposition of Gold Nanostructures onto Glassy Carbon Electrodes for Non-enzymatic Detection of Glucose. Electrochimica Acta, 132, pp. 524–532. doi.org/10.1016/j.electacta.2014.04.031.
- Ramanaviciene, A., et al. (2021) Glucose Biosensor Based on Dendritic Gold Nanostructures Electrodeposited on Graphite Electrode by Different Electrochemical Methods. Chemosensors, 9, p. 188. doi.org/10.3390/chemosensors9080188.
- German, N., et al. (2015) The use of different glucose oxidases for the development of an amperometric reagentless glucose biosensor based on gold nanoparticles covered by polypyrrole. Electrochimica Acta, 169, pp. 326–333. doi.org/10.1016/j.electacta.2015.04.072.
- Kausaite-Minkstimiene, A., et al. (2017) Reagent-less amperometric glucose biosensor based on a graphite rod electrode layer-by-layer modified with 1,10-phenanthroline-5,6-dione and glucose oxidase. Talanta, 171, pp. 204–212. doi.org/10.1016/j.talanta.2017.04.047.
- Le, T. X. H., et al. (2016) Gold particles growth on carbon felt for efficient micropower generation in a hybrid biofuel cell. Electrochimica Acta, 219, pp. 121–129. doi.org/10.1016/j.electacta.2016.09.135.
- Seker, E., et al. (2009) Nanoporous Gold: Fabrication, Characterization, and Applications. Materials, 2, pp. 2188–2215. https://doi.org/10.3390/ma2042188.
- Xu, M., et al. (2017) Kinetically controlled synthesis of nanoporous Au and its enhanced electrocatalytic activity for glucose-based biofuel cells. Nanoscale, 9, pp. 2514–2520. doi.org/10.1039/C6NR08518K.
- Huo, W. S., et al. (2017) Performance of glucose/O2 enzymatic fuel cell based on supporting electrodes over-coated by polymer-nanogold particle composite with entrapped enzymes. Chemical Physics Letters, 671, pp. 15–20. doi.org/10.1016/j.cplett.2017.01.005.
- Gholami, F., et al. (2018) Direct Enzymatic Glucose/O2 Biofuel Cell based on Poly-Thiophene Carboxylic Acid alongside Gold Nanostructures Substrates Derived through Bipolar Electrochemistry. Scientific Reports, 8, p. 15103. doi.org/10.1038/s41598-018-32893-2.
- Jayapiriya, U S & Goel, S (2020) Flexible and optimized carbon paste electrodes for direct electron transfer-based glucose biofuel cell fed by various physiological fluids. Applied Nanoscience, 10, pp. 4315–4324. doi.org/10.1007/s13204-020-01543-3.
- Zhang, X., et al. (2004) Polyelectrolyte Multilayer as Matrix for Electrochemical Deposition of Gold Clusters: Toward Super-Hydrophobic Surface. Journal of the American Chemical Society, 126, pp. 3064–3065. doi.org/10.1021/ja0398722.
- Yi, S., et al. (2013) One-step synthesis of dendritic gold nanoflowers with high surface-enhanced Raman scattering (SERS) properties. RSC Advances, 3, pp. 10139–10144. hdoi.org/10.1039/C3RA40716K.
- Huang, T., et al. (2010) Controlled Synthesis of Dendritic Gold Nanostructures Assisted by Supramolecular Complexes of Surfactant with Cyclodextrin. Langmuir, 26, pp. 7582–7589. doi.org/10.1021/la904393n.
- Shanmugam, M & Kim, K (2016) Electrodeposited gold dendrites at reduced graphene oxide as an electrocatalyst for nitrite and glucose oxidation. Journal of Electroanalytical Chemistry, 776, pp. 82–92. doi.org/10.1016/j.jelechem.2016.06.009.
- Hu, Y., et al. (2007) Fabrication of dendrite-like Au nanostructures and their enhanced photoluminescence emission. Physica Status Solidi, 204, pp. 3398–3404. doi.org/10.1002/pssa.200723022.
- Gustafsson, H., et al. (2015) Co-immobilization of enzymes with the help of a dendronized polymer and mesoporous silica nanoparticles. Journal of Materials Chemistry B, 3, pp. 6174–6184. doi.org/10.1039/C5TB00543D.
- Kausaite-Minkstimiene, A., et al. (2020) Reagent-less amperometric glucose biosensor based on nanobiocomposite consisting of poly(1,10-phenanthroline-5,6-dione), poly(pyrrole-2-carboxylic acid), gold nanoparticles and glucose oxidase. Microchemical Journal, 154, p. 104665. doi.org/10.1016/j.microc.2020.104665.
- Gooding, J. J., et al. (2001) Parameters Important in Fabricating Enzyme Electrodes Using Self-Assembled Monolayers of Alkanethiols. Analytical sciences, 17, pp. 3–9. doi.org/10.2116/analsci.17.3.
- German, N., et al. (2010) Glucose biosensor based on graphite electrodes modified with glucose oxidase and colloidal gold nanoparticles. Microchimica Acta, 168, pp. 221–229. doi.org/10.1007/s00604-009-0270-z
- Ameku, W. A., et al. (2019) Gold Nanoparticle Paper-Based Dual-Detection Device for Forensics Applications. ACS Applied Nano Materials, 2, pp. 5460–5468. doi.org/10.1021/acsanm.9b01057.
- Popov, A., et al. (2020) Electrodeposited Gold Nanostructures for the Enhancement of Electrochromic Properties of PANI–PEDOT Film Deposited on Transparent Electrode. Polymers, 12, p. 2778. doi.org/10.3390/polym12122778.
- Ramanavicius, A., et al. (2017) Evaluation of electron transfer in electrochemical system based on immobilized gold nanoparticles and glucose oxidase.Journal of the Electrochemical Society,164, pp. G45–G49.
- Nikolaev, K., et al. (2015) A novel bioelectrochemical interface based on in situ synthesis of gold nanostructures on electrode surfaces and surface activation by Meerwein’s salt. A bioelectrochemical sensor for glucose determination. Bioelectrochemistry, 105, pp. 34–43. doi.org/10.1016/j.bioelechem.2015.05.004.
- Sadak, O (2021) One-pot scalable synthesis of rGO/AuNPs nanocomposite and its application in enzymatic glucose biosensor. Nanocomposites, 7, pp. 44–52. doi.org/10.1080/20550324.2021.1917837.
- Zhang, S., et al. (2005) Covalent attachment of glucose oxidase to an Au electrode modified with gold nanoparticles for use as glucose biosensor. Bioelectrochemistry, 67, pp. 15–22. doi.org/10.1016/j.bioelechem.2004.12.002.
- Xiao, X., et al. (2014) Examining the effects of self-assembled monolayers on nanoporous gold based amperometric glucose biosensors. Analyst, 139, pp. 488–494. doi.org/10.1039/C3AN01670F.
- Yang, W., et al. (2006) Multilayered construction of glucose oxidase and gold nanoparticles on Au electrodes based on layer-by-layer covalent attachment. Electrochemistry Communications, 8, pp. 665–672. doi.org/10.1016/j.elecom.2005.11.014.
- German, N., et al. (2017) Amperometric Glucose Biosensor Based on Electrochemically Deposited Gold Nanoparticles Covered by Polypyrrole. Electroanalysis, 29, pp. 1267–1277. doi.org/10.1002/elan.201600680.