Over the years, atomic force microscopy, or AFM, has become a de facto standard in the high-resolution structural study of samples spanning from single molecules to complex macromolecular systems [1, 2].
AFM is different from other high-resolution imaging methods and does not need any particular sample to be modified (except for surface deposition). Hence, the risk of introducing artifacts through sample preparation is decreased.
In the past, there has been a huge demand for quantitative nanomechanical characterization of samples, application of innovative developments featuring high-speed AFM imaging (more than 10 frames/second), and cutting-edge feedback imaging modes, which tweak the highest achievable resolution.
In addition, the analysis of, for example, particular features or molecules carrying immunochemical data has been achieved by integrating AFM with the latest development in fluorescence/optical microscopy. This advancement complements the benefits of both methods and allows true correlative microscopy [3].
Correlative and High-Speed AFM
The measurements demonstrated in this article were made using the newly launched JPK NanoWizard® ULTRA Speed 2 with Bruker Nano’s second-generation Vortis™ controller — an industry standard in correlative, high-speed BioAFM integrated with sophisticated optical microscopy.
The instrument includes user-friendly modes, like QI™, which enables reliable and reproducible imaging of delicate and very soft samples in liquid. QI™ also helps in quantifying adhesive and mechanical sample characteristics with nanometer resolution.
HyperDrive™ is a more sophisticated mode that enables topography imaging of fragile and tiny structures, like single molecules in liquid, at the highest resolution.
This article illustrates the potential of the instrument to non-destructively analyze DNA nanostructures at 400 lines/second in closed-loop mode. Figure 1 shows a schematic depiction of a standard instrumental setup for the sophisticated correlative measurements used here.
.jpg)
Figure 1. JPK NanoWizard® AFM family integrated with a compact line STEDYCON (Abberior Instruments), on an AxioObserver (Zeiss).
STED — A Super-Resolution Microscopy Technique
In recent years, an array of super-resolution microscopy methods has been developed, and each of these methods proposes different ideas for resolving the Abbe diffraction limit. The minimum resolvable distance between two point-like objects is defined by the Abbe diffraction limit [4]. In an optical microscope, the resolution ends at about 600 nm axially and 200 nm laterally.
In 1994, Hell and Wichmann devised a novel technique to break the diffraction limit, stimulated emission depletion (STED), which received the Nobel Prize in Chemistry in 2014 [5]. Two laser pulses are used by STED microscopy. The first laser pulse excites, while the second one de-excites the fluorophores — fluorescent chemical compounds that re-emit light when stimulated by light.
While the excitation laser focus scans the sample, the depletion donut-shaped STED beam turns off the fluorophores at the edge of the focal point and confines the spot of effective fluorescence (see Figure 2).
.jpg)
Figure 2. Principle of combined AFM and STED microscopy. The “donut” shape of the encircling depletion (STED) laser confines the spot of effective fluorescence.
Boosting the intensity of the STED beam lowers the full width at half maximum (FWHM) of the effective focal spot dSTED as illustrated below:
.jpg)
λ indicates the wavelength, α is the half-aperture angle of the objective, n is the refractive index of the medium, I indicates the reduction in STED beam intensity, and Is is the typical saturation intensity for a specific fluorophore at which the emitted fluorescence is decreased by a factor of 1⁄e.
DNA as a Nanoscale Building Block
Deoxyribonucleic acid (DNA) is a polymer that is popular among chemists. The innate structure of the DNA is based on four organic bases that include a pair of alpha-helical strands held together by hydrogen bonds [6]. After a specific complementarity code, the sequences in the alpha-helical strands bind to each other and present many possibilities for (non)-directed self-assembly into complex structures and shapes on the nanoscale [7].
In a DNA strand, the sequence governs the way it assembles and this helps in predicting or modeling the final structure that will be adopted by a DNA molecule in vitro. When specific sequences are used, one or more DNA strands can be made to assemble into complex architectures. Seeman laid the groundwork for manipulating DNA for nanotechnology and illustrated a number of approaches for the development of complex DNA structures [8].
New design strategies are being developed and these, in turn, are increasing the diversity of structures and their uses in various fields. For example, Ackermann et al. presented rotaxanes created from DNA, paving the way for research into synthetic biology and molecular machines [9, 10]. Benson and team illustrated an automated method for changing digitally created flat sheet meshes into scaffolded DNA structures [11].
DNA-Based Nanorulers
The use of DNA origami structures as calibration standards for 3D and 2D super-resolution optical microscopes is yet another application [12–14]. Commercial DNA-based rulers, used for quantifying and calibrating super-resolution systems and with an accuracy of just a few nanometers, come in many different forms with different fluorescence tags, fluorophore spacings, and nanostructure size and shape [15].
In order to illustrate the compatibility of these structures for correlative AFM measurements, commercially available STED nanorulers (GATTAquant GmbH) were utilized. These nanorulers are rod-shaped and altered with dye molecules located at defined distances along the rod (see Figure 3 a, b).
![Correlative AFM of DNA Nanorulers. (a, b) are STED measurements of the corresponding SIM 160R and STED 70R nanorulers, measured in TAE-1x (Mg) buffer. Insets are sketches of GATTAquant nanorulers with different mark-to-mark distances (70–160 nm, both labeled with Atto647N), reproduced with permission from [15]. (c, d) show bi-sigmoidal Gaussian fits of the intensity signal along the signified cross-sections in (a, b). (e, f) Optical correlation of the consecutively acquired STED and AFM images of the DNA nanorulers. (g, h) QI topography channels of the AFM images used for the overlays in (e, f), showing the linearized structure of the DNA nanorulers (z-scale: 10 nm).](https://www.azonano.com/images/Article_Images/ImageForArticle_5301(3).jpg)
Figure 3. Correlative AFM of DNA Nanorulers. (a, b) are STED measurements of the corresponding SIM 160R and STED 70R nanorulers, measured in TAE-1x (Mg) buffer. Insets are sketches of GATTAquant nanorulers with different mark-to-mark distances (70–160 nm, both labeled with Atto647N), reproduced with permission from [15]. (c, d) show bi-sigmoidal Gaussian fits of the intensity signal along the signified cross-sections in (a, b). (e, f) Optical correlation of the consecutively acquired STED and AFM images of the DNA nanorulers. (g, h) QI topography channels of the AFM images used for the overlays in (e, f), showing the linearized structure of the DNA nanorulers (z-scale: 10 nm).
DNA-Based Origami
In some scenarios, it is beneficial to use both an AFM and optical calibration standard. In this study, a new rectangular origami (NRO) design having 100 x 70 nm dimensions was used. [14] Structures like those are available in the market as GATTA-AFM. In such a case, these structures selectively carry a pair of diagonally spaced Atto647N fluorophore populations (Figure 4a, inset).
![Characterization of GATTA-AFM rectangular origami. (a-inset) Sketch of а DNA rectangular origami (GATTA-AFM) with the theoretical locations for the Atto647N fluorophores, reproduced with permission from [15]. (a) STED measurement of the corresponding signal from DNA rectangles carrying a fluorophore and measured in TAE-1x (Mg) buffer. (b) Fast amplitude modulation (AM) AFM image of a DNA origami lattice (without a fluorophore) recorded at 10 lines/second. The inset represents a cross-section across the central ladder seam of the DNA nanostructure (z-scale: 2 nm). (c) Fast phase modulation (PM) AFM image of a DNA origami lattice carrying Atto647N labels, recorded at 10 lines/second. The inset signifies the locations along the lattice, which are supposedly carrying the fluorescent tags (z-scale: 3 nm).](https://www.azonano.com/images/Article_Images/ImageForArticle_5301(4).jpg)
Figure 4. Characterization of GATTA-AFM rectangular origami. (a-inset) Sketch of а DNA rectangular origami (GATTA-AFM) with the theoretical locations for the Atto647N fluorophores, reproduced with permission from [15]. (a) STED measurement of the corresponding signal from DNA rectangles carrying a fluorophore and measured in TAE-1x (Mg) buffer. (b) Fast amplitude modulation (AM) AFM image of a DNA origami lattice (without a fluorophore) recorded at 10 lines/second. The inset represents a cross-section across the central ladder seam of the DNA nanostructure (z-scale: 2 nm). (c) Fast phase modulation (PM) AFM image of a DNA origami lattice carrying Atto647N labels, recorded at 10 lines/second. The inset signifies the locations along the lattice, which are supposedly carrying the fluorescent tags (z-scale: 3 nm).
Figure 4a shows the STED signal emitted from such structures. The molecular substructure of these conformations can be easily identified in AFM images because of their planar design and two typical features of their pattern (see Figure 4c). The first is the middle ladder seam that bridges the crossed halves of the design, which has a 6-nm pitch, as reported in Gattaquant DNA Nanotechnologies [15].
Secondly, six visible gaps exist in the NRO lattice (marked here with circles) and these are introduced through shortened staple strands, as labeled binding sites for other molecules [14].
Phase-modulation AFM (HyperDrive™) was used to examine the NRO design carrying fluorophores, and this is illustrated in Figure 4c. Each binding locus (20 x 20 nm) on the lattice is hybridized by 12–15 ssDNA, according to the manufacturer. Although these locations are evidently seen at each end, they show a certain hydrodynamic radius fluctuation because of their length (25 bp). Such a fluctuation inhibits a clear resolution of the NRO lattice.
High-Speed Imaging of DNA Origami
In the repertoire of AFM, high-speed applications are a continuous development and have been found to be specifically fascinating in the characterization of dynamic and kinetic processes. With regards to DNA origami, events like those can be either the (non-)assisted self-assembly of surface structures and lattices, or merely the binding of certain single molecules and cargo. Here, the NRO lattices (without fluorophores) were examined using high-speed AFM (see Figure 5).
.jpg)
Figure 5. High-speed AFM images of R80 DNA Origami. Both images (256 x 256 pixels) were recorded at 400 lines/second, resulting in a temporal resolution of ~1.6 frames/second. Z-scale in both images is 2 nm.
The resulting AFM images reveal that NRO’s molecular structures can be non-invasively studied at a line rate of 400 lines/second and with an image resolution of 256 x 256 pixels. Moreover, the temporal resolution of 1.6 frames/second (fps) can be additionally increased to more than 10 fps by using the bi-directional scanning implemented in the NanoWizard® ULTRA Speed 2, and/or by reducing the line resolution. Consecutively, this would help in resolving dynamic events that occur on the millisecond scale.
Conclusion
The article shows how the advanced JPK NanoWizard® ULTRA Speed 2 AFM was successfully used for analyzing DNA-based nanostructures with sub-molecular resolution in vitro.
It also demonstrated that the instrument can be effectively combined with a 2D compact line super-resolution STED instrument and can non-invasively perform correlative microscopy with better than 70 nm lateral resolution. In addition, the potential of this setup to examine dynamic processes with a temporal resolution of more than 1 fps was also illustrated.
References
- Stamov DR, Kaemmer SB, Hermsdörfer A, Barner J, Jähnke T, Haschke H (2015) BioScience AFM – Capturing Dynamics from Single Molecules to Living Cells. Microsc Today 23:18–25. doi: 10.1017/S1551929515001005
- Winkel AK, Barner J, Henze T, Neumann T, Körnig A, Kumpfe F, Haschke H (2016) The Wide-Open Door: Atomic Force Microscopy 30 Years On. Microsc Today 24:12–17. doi: 10.1017/S1551929516000900
- Monserrate A, Casado S, Flors C (2014) Correlative Atomic Force Microscopy and Localization-Based Super-Resolution Microscopy: Revealing Labelling and Image Reconstruction Artefacts. ChemPhysChem 15:647–650. doi: 10.1002/cphc.201300853
- Endesfelder U (2018) Super-Resolution Microscopy. A Practical Guide. By Udo J. Birk. Angew Chem Int Ed 57:7939–7939. doi: 10.1002/anie.201804434
- Hell SW, Wichmann J (1994) Breaking the diffraction resolution limit by stimulated emission: stimulated-emission-depletion fluorescence microscopy. Opt Lett 19:780–782. doi: 10.1364/OL.19.000780
- Watson JD, Crick FHC (1953) Molecular Structure of Nucleic Acids: A Structure for Deoxyribose Nucleic Acid. Nature 171:737–738. doi: 10.1038/171737a0
- Sanderson K (2010) Bioengineering: What to make with DNA origami. Nature 464:158–159. doi: 10.1038/464158a
- Seeman NC (1998) DNA NANOTECHNOLOGY: Novel DNA Constructions. Annu Rev Biophys Biomol Struct 27:225–248. doi: 10.1146/annurev.biophys.27.1.225
- Ackermann D, Schmidt TL, Hannam JS, Purohit CS, Heckel A, Famulok M (2010) A double-stranded DNA rotaxane. Nat Nanotechnol 5:436–442. doi: 10.1038/nnano.2010.65
- Ackermann D, Jester S-S, Famulok M (2012) Design Strategy for DNA Rotaxanes with a Mechanically Reinforced PX100 Axle. Angew Chem Int Ed 51:6771–6775. doi: 10.1002/anie.201202816
- Benson E, Mohammed A, Bosco A, Teixeira AI, Orponen P, Högberg B (2016) Computer-Aided Production of Scaffolded DNA Nanostructures from Flat Sheet Meshes. Angew Chem Int Ed 55:8869–8872. doi: 10.1002/anie.201602446
- Steinhauer C, Jungmann R, Sobey T, Simmel F, Tinnefeld P (2009) DNA Origami as a Nanoscopic Ruler for Super-Resolution Microscopy. Angew Chem Int Ed 48:8870–8873. doi: 10.1002/anie.200903308
- Schmied JJ, Forthmann C, Pibiri E, Lalkens B, Nickels P, Liedl T, Tinnefeld P (2013) DNA Origami Nanopillars as Standards for Three-Dimensional Superresolution Microscopy. Nano Lett 13:781–785. doi: 10.1021/nl304492y
- Schmied JJ, Raab M, Forthmann C, Pibiri E, Wünsch B, Dammeyer T, Tinnefeld P (2014) DNA origami–based standards for quantitative fluorescence microscopy. Nat Protoc 9:1367–1391. doi: 10.1038/nprot.2014.079
- Gattaquant DNA Nanotechnologies. In: Gattaquant DNA Nanotechnologies. http://www.gattaquant.com/. Accessed 28 Jan 2019
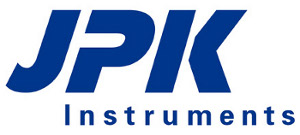
This information has been sourced, reviewed and adapted from materials provided by JPK Instruments AG - Scanning Probe Technologies.
For more information on this source, please visit JPK Instruments AG - Scanning Probe Technologies.