Dry reforming of methane (DRM) has been demonstrated to help in greenhouse gas mitigation. However, a proper catalyst is required for catalytic activity and stability during DRM. Research published in the MDPI journal molecules investigated the effect of strontium incorporation into cerium- and nickel-based nanocrystalline perovskites for greenhouse gas mitigation strategies.
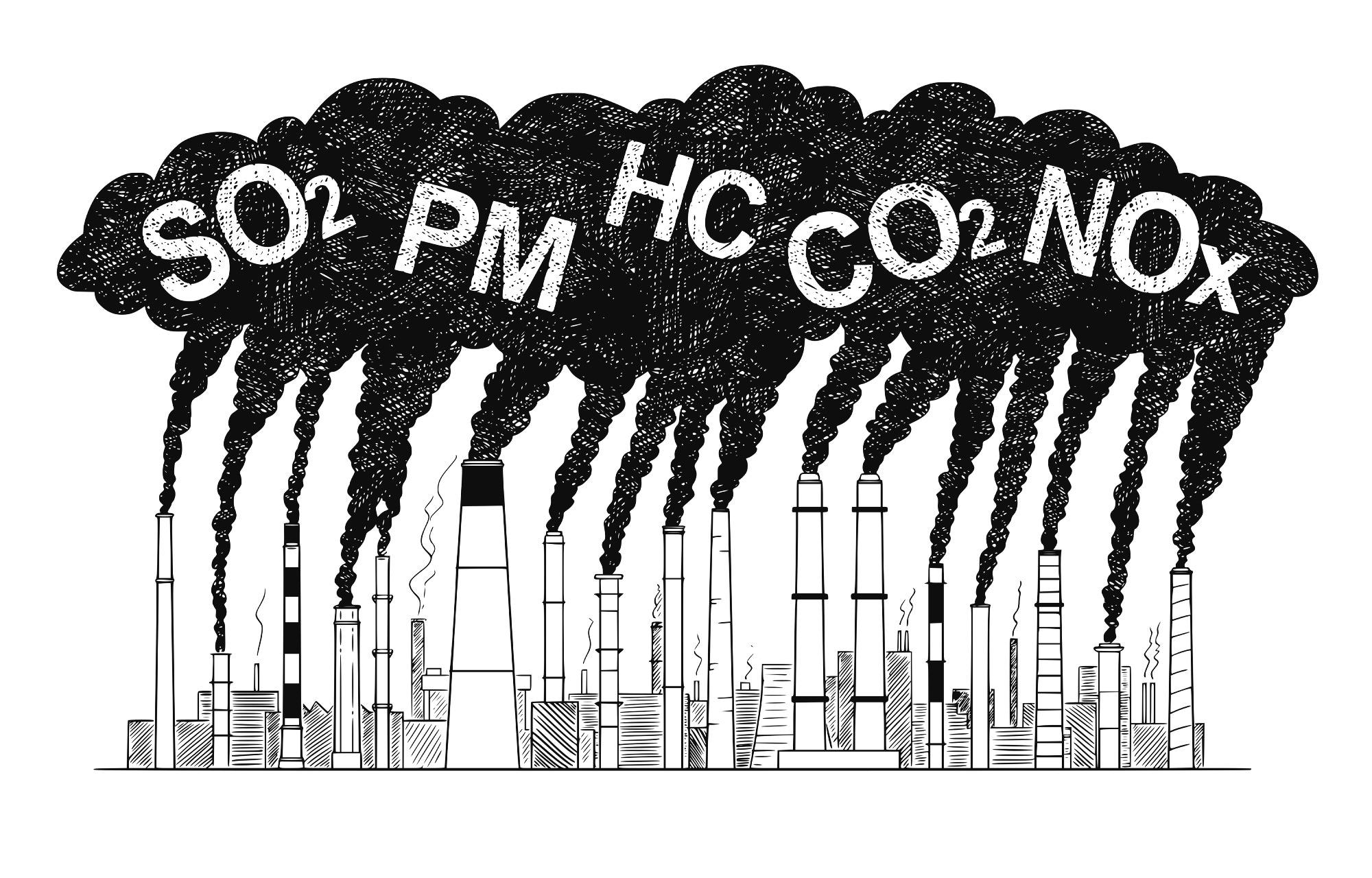
Image Credit: Zdenek Sasek/Shutterstock.com
Dry reforming of methane (DRM), among the other CO2 conversion, has garnered attention majorly because DRM converts major greenhouse gases—methane and carbon dioxide—to produce hydrogen and carbon monoxide, which is then used to produce liquid hydrocarbons.
The DRM process plays a role in greenhouse gas mitigation and serves as a cause of climate change. However, it also produces synthesis gas, a mixture of equimolar hydrogen and carbon monoxide appropriate for the synthesis of hydrocarbon with the help of the Fischer–Tropsch synthesis process.
Both transition metal-based and noble metal-based catalysts are utilized, but transition metal-based catalysts like Nickel (Ni)- and cobalt (Co)-based catalysts are most analyzed for DRM as they are inexpensive, abundant, and provide quick turnover rates. Ni-based catalysts become deactivated during DRM, a major challenge to be overcome.
Metallic nanoparticles supported on basic oxide supports or promoters, show enhanced catalytic activity and benefit CO2 chemisorption. Nickel-based double-layered hydroxides (LDHs), in addition to the metallic nanoparticle-supported oxides, have demonstrated better catalytic performances towards DRM reaction. However, they eventually deactivate owing to the rapid growth of randomly sized impregnated nanoparticles.
The current study shows the effect of incorporation of strontium (Sr) into cerium- and nickel-based nanocrystalline perovskites (CeNiO3) in both dry and/or CO2 reforming of methane. The research concentrates on analyzing the insights of the replacement of cerium with strontium and the impact of this replacement on catalytic conversions and durability.
Results
The thermal decomposition curves, which include thermogravimetric (TG) and the differentials (DTG) of the precursors as a measurement of their calcination temperatures, are depicted in Figure 1. It can be observed that each precursor decomposed differently.
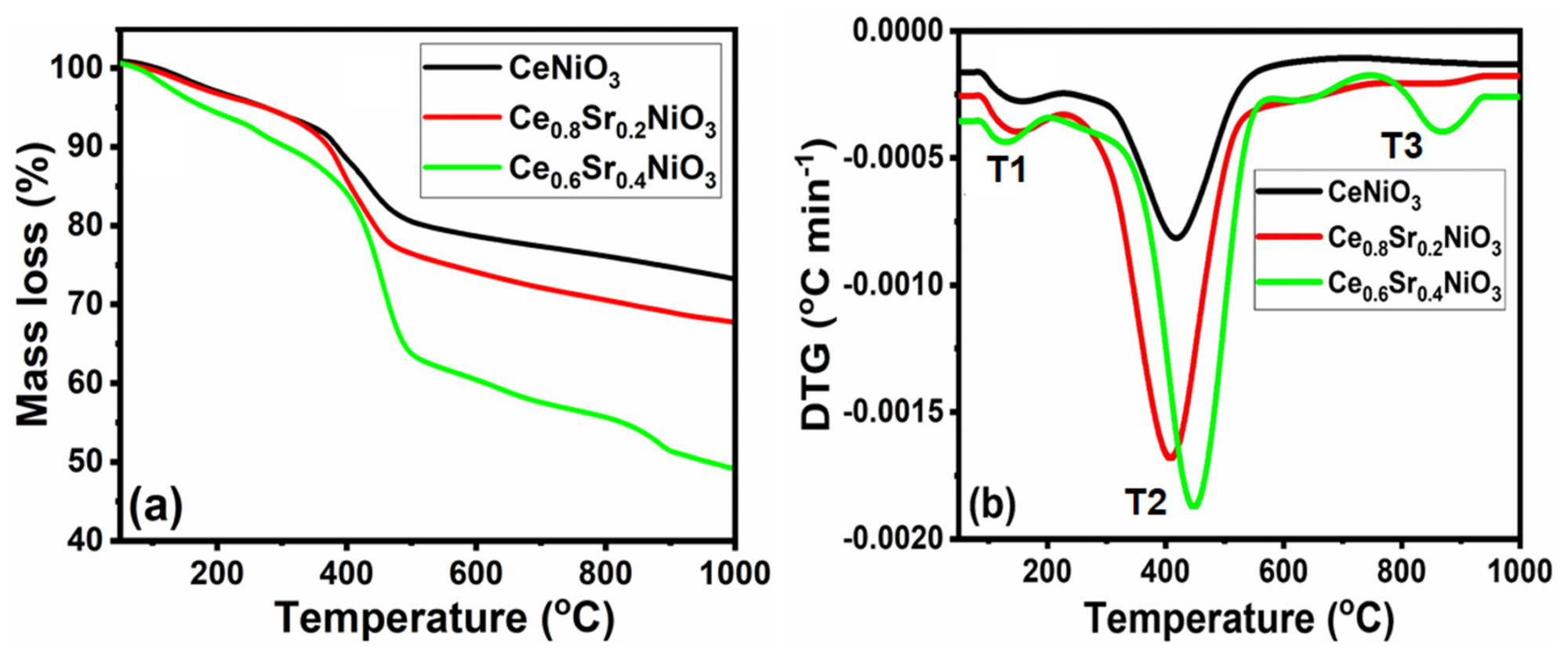
Figure 1. TG−DTG curves versus temperature of CexSr1−xNiO3 (x = 0.6–1) perovskites. Image Credit: Ahmad, et al., 2022
The XRD patterns of the as-prepared nanocrystalline perovskites without and with the incorporation are illustrated in Figure 2. MDI Jade® software was employed to analyze XRD data and a substantial number of peaks corresponding to CeNiO3 were recorded.
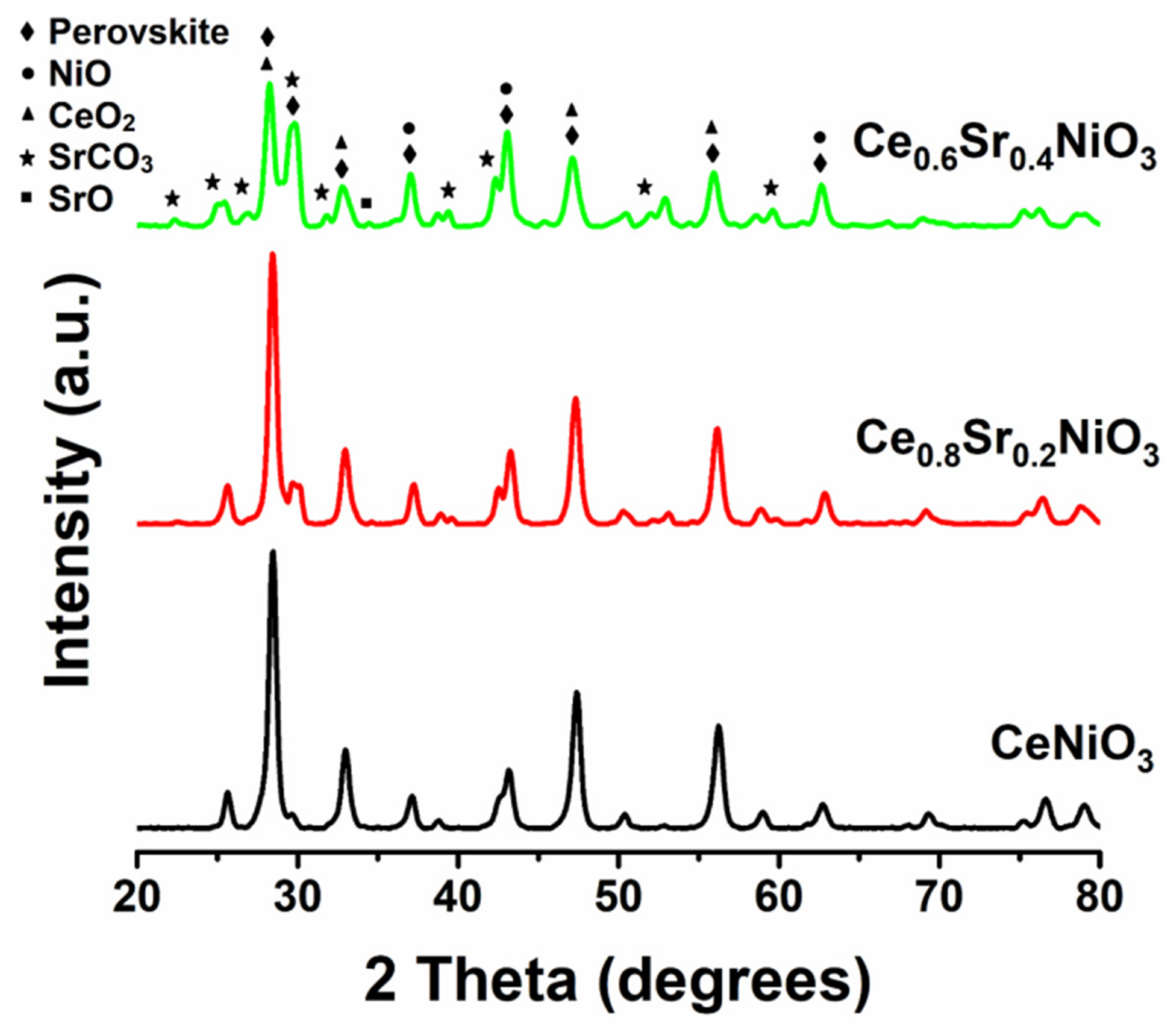
Figure 2. XRD patterns of CexSr1−xNiO3 (x = 0.6–1) perovskites. Image Credit: Ahmad, et al., 2022
Figure 3 reveals the N2 adsorption–desorption isotherms and the pore-size distributions of air-calcined precursors (700 °C, 5 hours). Figure 3 depicts that all of the samples showed a type II isotherm with an H3 hysteresis loop, indicating that these samples had macro-pores.
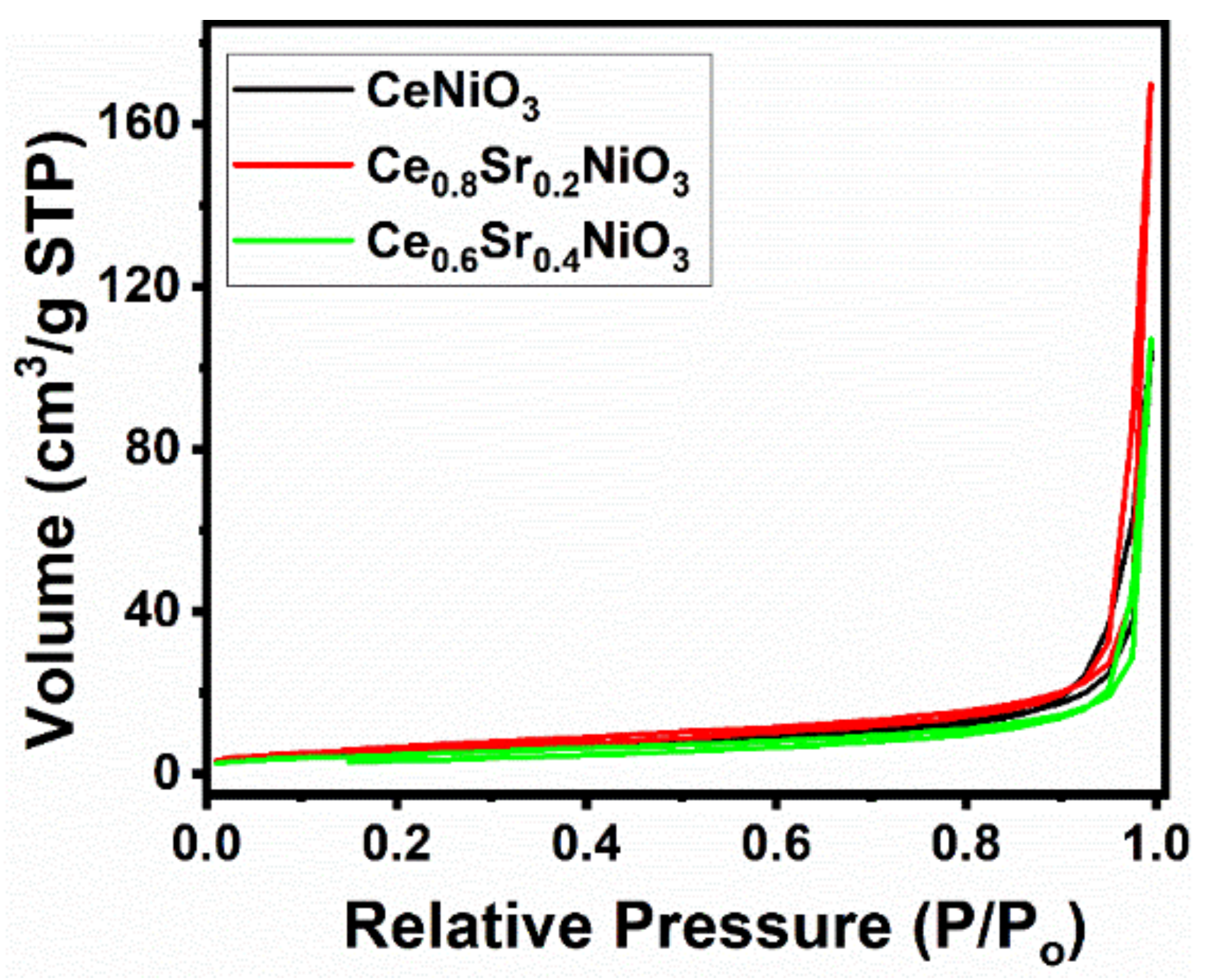
Figure 3. N2 adsorption–desorption isotherms of CexSr1−xNiO3 (x = 0.6–1) perovskites. Image Credit: Ahmad, et al., 2022
The BET (Braunner, Emmet, and Teller) surface areas and pore parameters are listed in Table 1.
Table 1. Textural properties and deactivation factors of CexSr1−xNiO3(x = 0.6–1) perovskites. Source: Ahmad, et al., 2022
Perovskites |
Ni/Ce/Sr Content (%) a |
SBET
(m2/g) |
Pore Volume
(cm3/g) |
Pore size
(nm) |
Deactivation
Factor (%) |
Coke (wt%) d |
Fresh |
Used |
CeNiO3 |
49.8/50.1/- |
50.2/49.6/- |
20.7 |
0.162 |
30.1 |
7.7 b (0.88) c |
9.1 |
Ce0.8Sr0.2NiO3 |
51.1/44.1/4.7 |
50.9/43.8/5.3 |
25.6 |
0.261 |
40.8 |
−62.6 b (1.71) c |
4.7 |
Ce0.6Sr0.4NiO3 |
50.7/32.1/17.2 |
51/31.1/17.8 |
17.3 |
0.164 |
38.1 |
−13.4 b (1.15) c |
2.1 |
a Determined from ICP-OES. b Deactivation factor (D.F., %) = 100 × (CH4conversioninitial − CH4conversionfinal)/(CH4conversioninitial). c D.F based on first-order deactivation, % = ln(1 − CH4conversionfinal)/ln(1 − CH4conversioninitial). d Calculated from TPO data.
The morphology of the nanocrystalline perovskite catalysts (fresh, reduced, and used) were analyzed using transmission electron microscopy (TEM). Figure 4 shows the images of the catalysts before and after the reaction. The particles were spherical in shape. Insignificant sintering was noted for reduced catalysts.
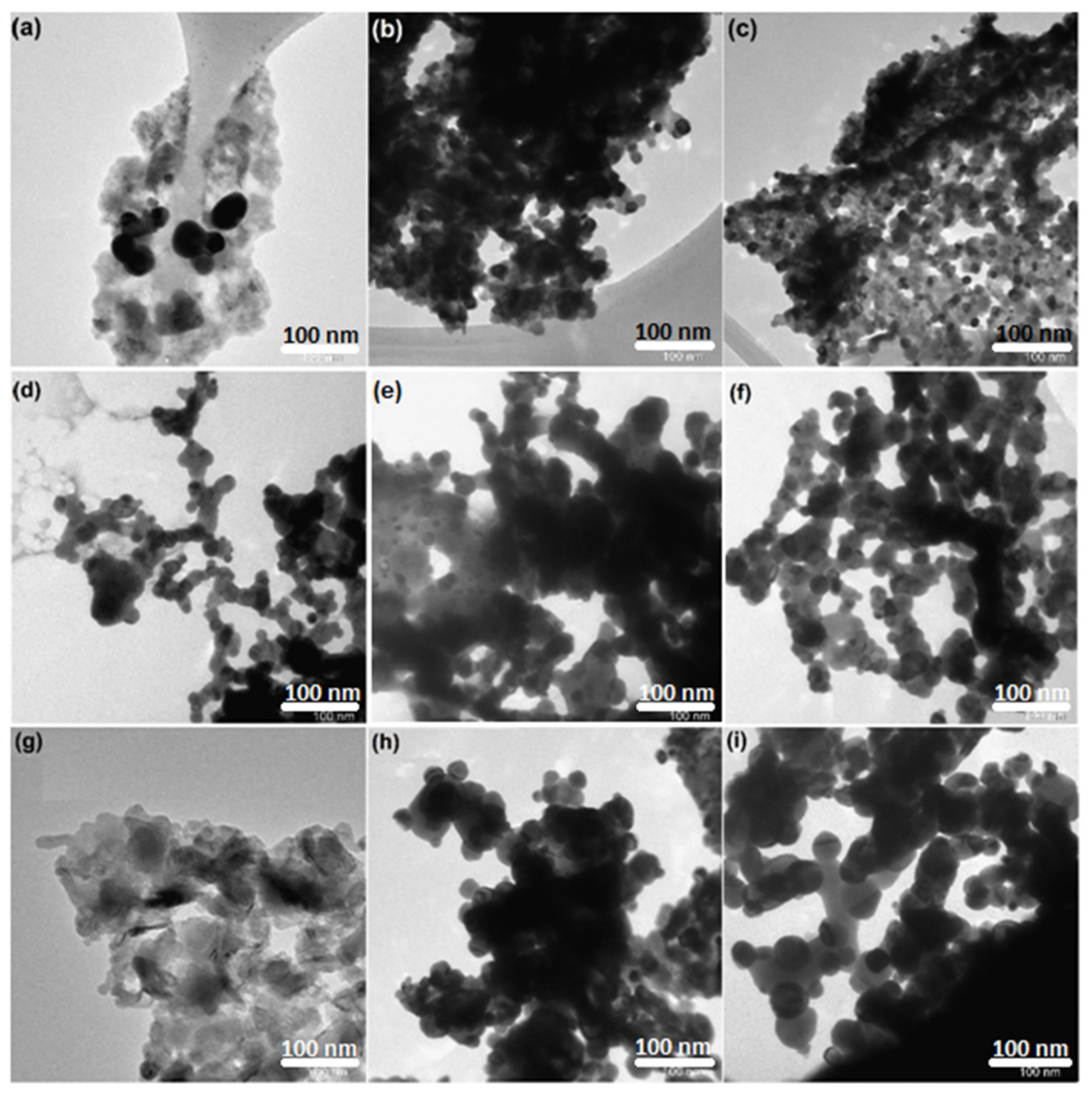
Figure 4. TEM images of CexSr1−xNiO3 (x = 0.6–1) perovskites; fresh catalysts: (a) CeNiO3, (b) Ce0.8Sr0.2NiO3, (c) Ce0.6Sr0.4NiO3; reduced catalysts: (d) CeNiO3, (e) Ce0.8Sr0.2NiO3, (f) Ce0.6Sr0.4NiO3; spent catalysts: (g) CeNiO3, (h) Ce0.8Sr0.2NiO3, (i) Ce0.6Sr0.4NiO3. Image Credit: Ahmad, et al., 2022
Temperature-Programmed Reduction (TPR) is majorly used to investigate the metal–support interaction, the reducibility, and to identify the activation and/or reduction temperature. Figure 5 shows the reduction profiles, denoting the differences in metal–support interaction and/or the reducibility of CeNiO3 after Sr addition.
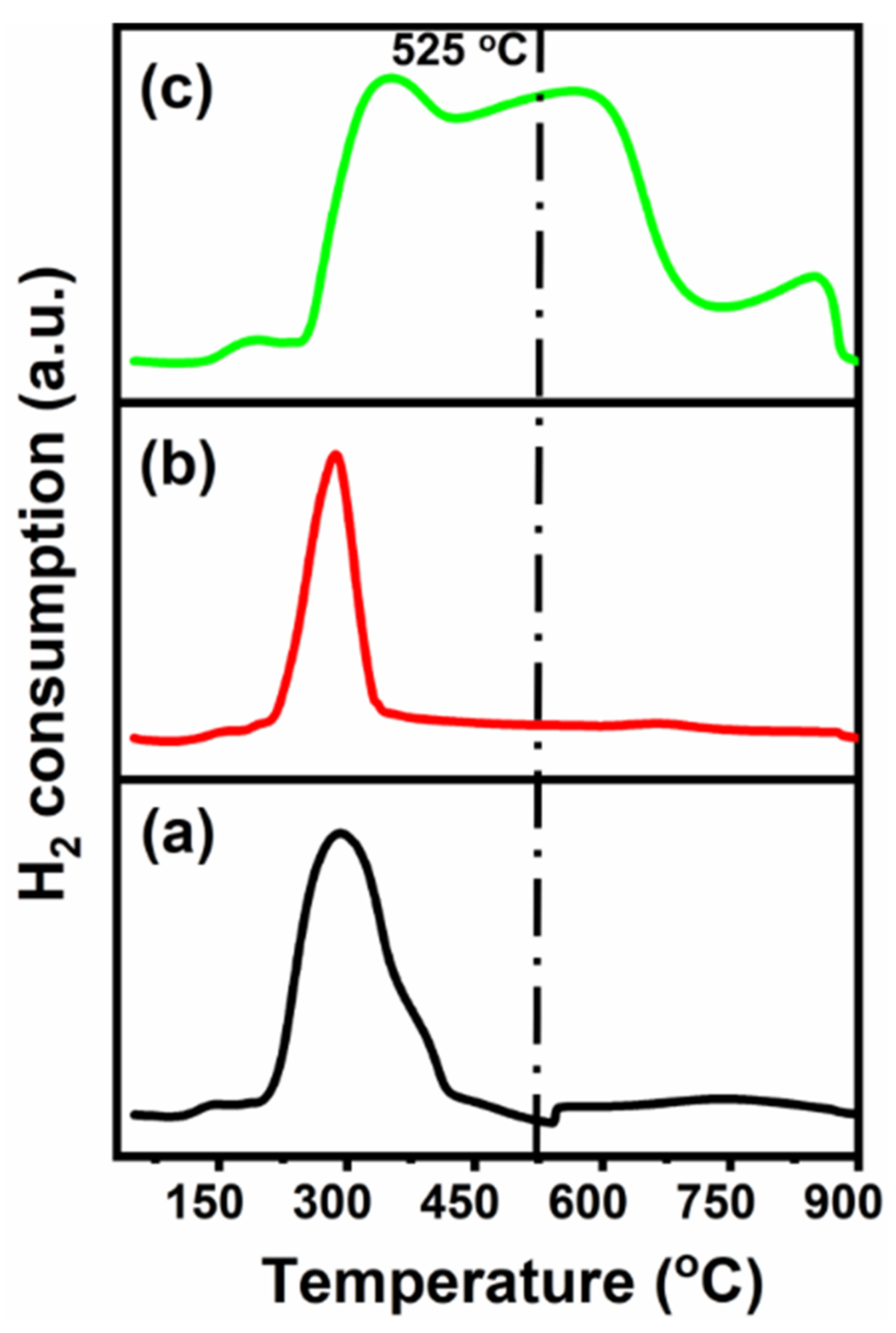
Figure 5. H2-TPR profiles of (a) CeNiO3, (b) Ce0.8Sr0.2NiO3, and (c) Ce0.6Sr0.4NiO3 perovskites. Image Credit: Ahmad, et al., 2022
The carbon dioxide (CO2) and methane (CH4) conversions versus time-on-stream are depicted in Figure 6. It was noted that CO2 conversions greater than CH4 conversions were responsible for side reactions including reverse water–gas-shift reaction and reverse CO disproportionation.
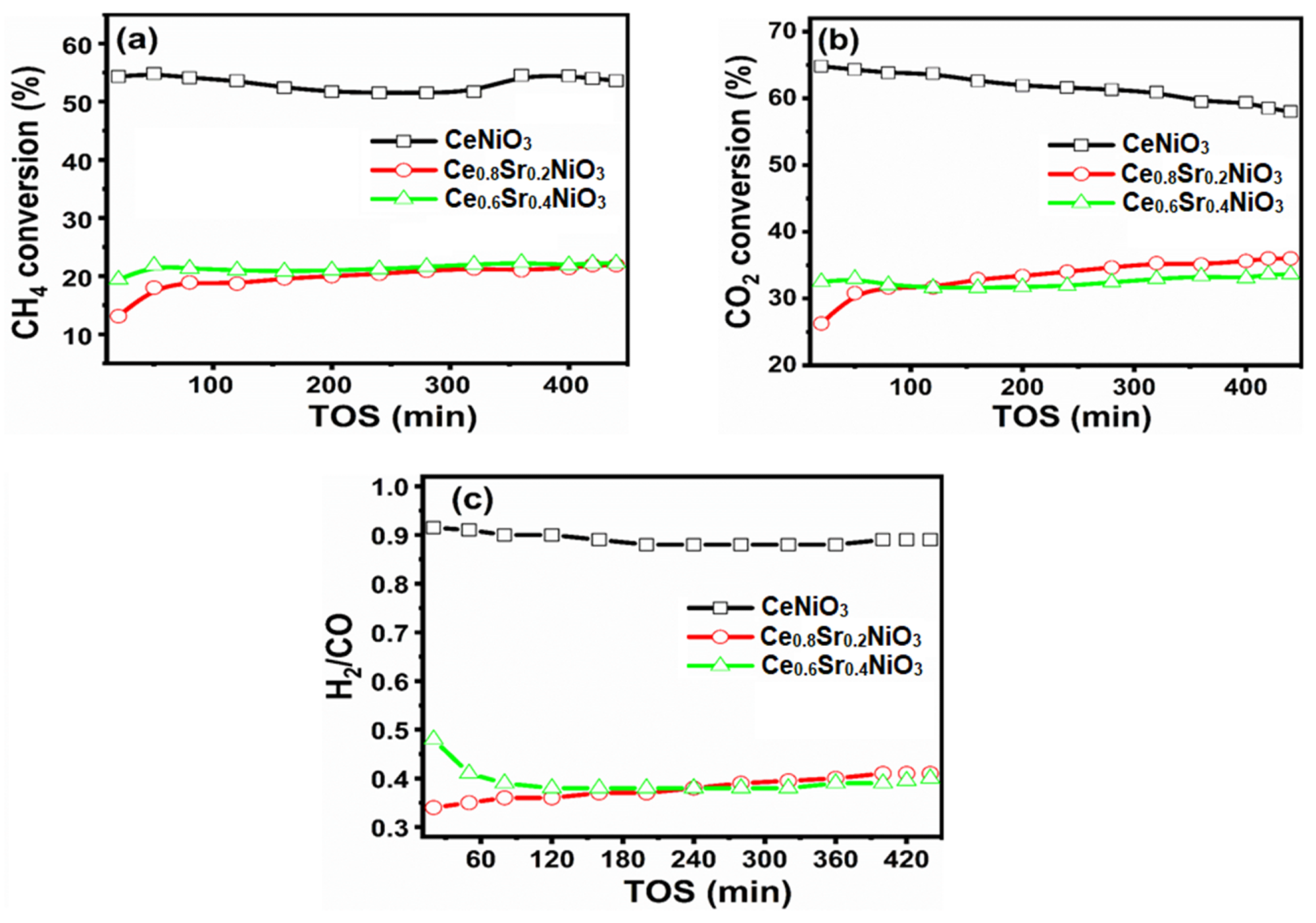
Figure 6. (a) CH4 conversion, (b) CO2 conversion, and (c) H2/CO ratios versus time-on-stream (TOS) of CexSr1−xNiO3 (x = 0.6–1) perovskites. Image Credit: Ahmad, et al., 2022
The likelihood of carbon formation over the catalyst’s surface after a dry-reforming reaction was shown by temperature-programmed oxidation (TPO) analysis (see Figure 7). A single broad peak was observed in the temperature range of 110 °C to 500 °C for each catalyst.
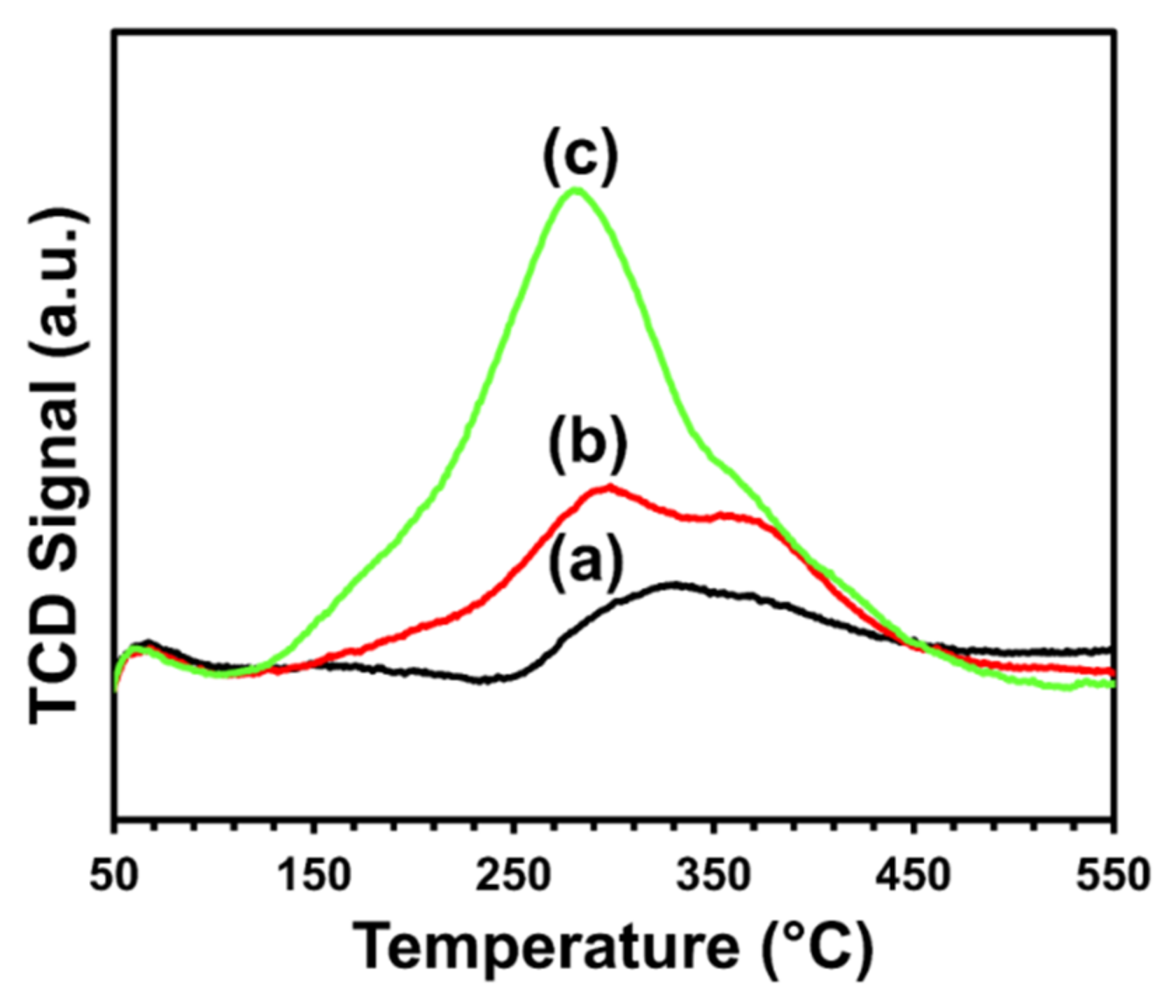
Figure 7. TPO profiles of (a) CeNiO3, (b) Ce0.8Sr0.2NiO3, and (c) Ce0.6Sr0.4NiO3 perovskites. Image Credit: Ahmad, et al., 2022
The TEM microscopic images were recorded after a dry-reforming reaction to comprehend the morphological changes and also the formation of deactivating coke above the surface of spent catalysts.
Discussion
Ahead of the dry methane-reforming reaction analysis, the as-prepared nanocrystalline perovskites were characterized. The mechanism connected with dry methane reforming needs reactant adsorption over the active sites of the catalyst. However, in spite of higher activity, CeNiO3 perovskite deactivated over time, these observations were substantiated by TEM and TPO.
But strontium-incorporated perovskites did not deactivate in spite of carbon deposition and sintering. The degree of agglomeration or sintering was the same for all perovskites. This indicates that methane decomposition, a prevalent DRM side reaction at the higher reaction temperature, is the major source of carbon deposition resulting in catalyst deactivation. Figure 8 illustrates the reaction mechanism and the impact of Sr incorporation.
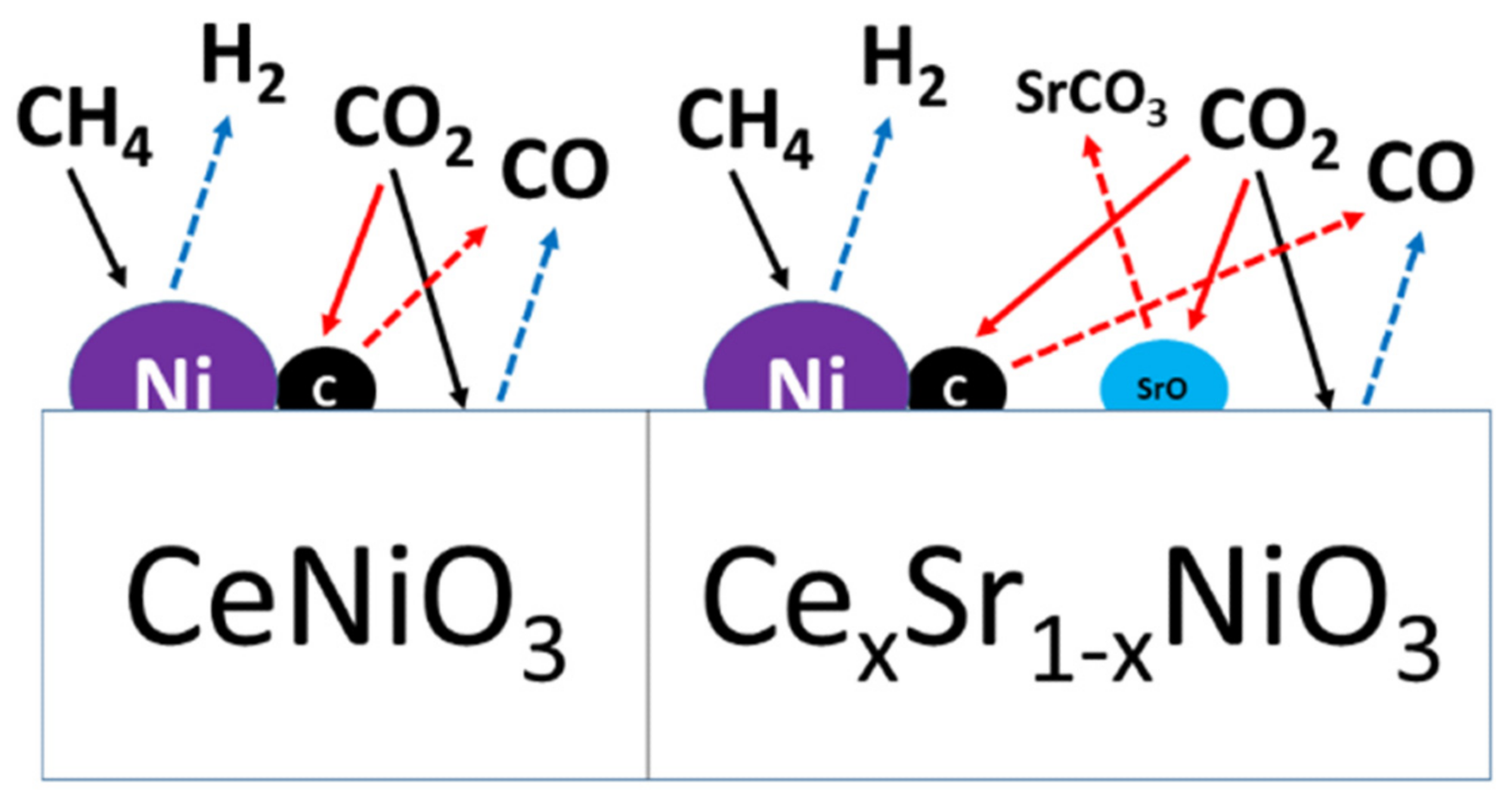
Figure 8. Schematic diagram of the reaction mechanism over Sr-free and Sr-incorporated perovskites. Image Credit: Ahmad, et al., 2022
Earlier studies inferred that Sr incorporation in lesser amounts showed less-active, but stable, catalytic performance, and Table 2 compares the observations of this study with earlier works. It was seen that the current conversions outperformed similar perovskites.
Table 2. Comparison of current work with previously reported work. DF refers to the deactivation factor. TOS indicates time-on-stream. Source: Ahmad, et al., 2022
Catalyst |
Reaction Temp. (°C)/
GHSV (L/h/gcat) |
Highest CH4
Conversion (%) |
%DF a |
TOS
(h) |
Ref. |
CeNiO3 |
750/72 |
32 |
12.9 |
~26 |
[55] |
La0.6Sr0.4NiO3 |
700/- |
85 |
- |
20 |
[56] |
La0.9Sr0.1NiO3 |
700/18 |
70 |
3.6 |
8 |
[57] |
LaNi0.8Fe0.2O3 |
800/13.7 |
65 |
7.7 |
35 |
[58] |
La0.5Sr0.5NiO3 |
750/18 |
69 |
47.8 |
24 |
[59] |
CeNiO3 |
700/84 |
55 |
7.7 |
8 |
This
work |
Ce0.8Sr0.2NiO3 |
22.5 |
−62.6 |
Ce0.6Sr0.4NiO3 |
22.5 |
−13.4 |
a Deactivation Factor (D.F., %) = 100 × (CH4conversioninitial − CH4conversionfinal)/(CH4conversioninitial).
Methodology
Nanocrystalline perovskites—CexSr1−xNiO3 (x = 0.6–1)—were produced through the self-combustion method. The thermogravimetric analysis (TGA) of the perovskite precursors was documented with a thermal analyzer. X-ray diffraction profiles were recorded and BET specific surface areas of the samples were evaluated.
The morphology of both fresh and used perovskite catalysts was examined by transmission electron microscopy, and TPO and TPR profiles were conducted. The as-synthesized catalysts’ chemical compositions were measured by inductively coupled plasma optical emission spectroscopy.
The dry methane-reforming reaction was carried out in a tubular fixed-bed reactor at 700 °C and 1 atm pressure. The feed gas was flown at 70 mL/min and the catalysts were subjected to activation/reduction, before the reaction. The hydrogen mixture was replaced with helium after activation, to remove any leftover hydrogen.
Conclusion
The current study showed the catalytic performance results of strontium-incorporated nanocrystalline perovskites for dry methane reforming. The observations reveal the major role of strontium incorporation in CexSr1−xNiO3 (x = 0.6–1) perovskites to ascertain stable catalytic performance for prolonged periods to avoid deactivation.
Journal Reference:
Ahmad, N., Wahab, R., Manoharadas, S., Alrayes, B. F., Alam, M., Alharthi, F. A., (2022) The Role of Strontium in CeNiO3 Nano-Crystalline Perovskites for Greenhouse Gas Mitigation to Produce Syngas. Molecules, 27(2), p.356. Available Online: https://www.mdpi.com/1420-3049/27/2/356/htm.
References and Further Reading
- Wang, Y., et al. (2018) Low-temperature catalytic CO2 dry reforming of methane on Ni-based catalysts: A review. Fuel Processing Technology, 169, pp. 199–206. doi.org/10.1016/j.fuproc.2017.10.007.
- Li, H., et al. (2017) Design an in-situ reduction of Ni/C–SiO2 catalyst and new insights into pretreatment effect for CH4–CO2 reforming reaction. International Journal of Hydrogen Energy, 42, pp. 10844–10853. doi.org/10.1016/j.ijhydene.2017.03.156.
- Al-Fatesh, A. S., et al. (2017) CO2-reforming of methane to produce syngas over Co-Ni/SBA-15 catalyst: Effect of support modifiers (Mg, La, and Sc) on catalytic stability. Journal of CO2 Utilization, 21, pp. 395–404. doi.org/10.1016/j.jcou.2017.08.001.
- Ibrahim, A., et al. (2014) Enhancing hydrogen production by dry reforming process with strontium promoter. International Journal of Hydrogen Energy, 39, pp. 1680–1687. doi.org/10.1016/j.ijhydene.2013.11.050.
- Li, D., et al. (2017) Preparation of supported Co catalysts from Co–Mg–Al layered double hydroxides for carbon dioxide reforming of methane. International Journal of Hydrogen Energy, 42, pp. 5063–5071. doi.org/10.1016/j.ijhydene.2016.10.114.
- Al-Fatesh, A. S., et al. (2018) Evaluation of Co-Ni/Sc-SBA–15 as a novel coke resistant catalyst for syngas production via CO2 reforming of methane. Applied Catalysis A: General, 567, pp. 102–111. doi.org/10.1016/j.apcata.2018.09.012.
- Rynkowski, J., et al. (2004) Catalytic performance of reduced La2−xSrxNiO4 perovskite-like oxides for CO2 reforming of CH4. Applied Catalysis A: General, 263, pp. 1–9. doi.org/10.1016/j.apcata.2003.11.022.
- El Hassan, N., et al. (2016) Low temperature dry reforming of methane on rhodium and cobalt based catalysts: Active phase stabilization by confinement in mesoporous SBA-15. Applied Catalysis A: General, 520, pp. 114–121. doi.org/10.1016/j.apcata.2016.04.014.
- Khan, W. U., et al. (2021) Copper-Promoted Cobalt/Titania Nanorod Catalyst for CO Hydrogenation to Hydrocarbons. Catalysis Letters, 151, pp. 2492–2501. doi.org/10.1007/s10562-020-03506-3.
- Khan, W. U., et al. (2021) Recent progress in CO hydrogenation over bimetallic catalysts for higher alcohol synthesis. ChemCatChem, 13, pp. 111–120. doi.org/10.1002/cctc.202001436.
- Khan, W. U., et al. (2020) Catalytically active interfaces in titania nanorod-supported copper catalysts for CO oxidation. Nano Research, 13, pp. 533–542. doi.org/10.1007/s12274-020-2647-6.
- Horn, R & Schlögl, R (2015) Methane Activation by Heterogeneous Catalysis. Catalysis Letters, 45, pp. 23–39. doi.org/10.1007/s10562-014-1417-z.
- Rostrup-Nielsen, J. R., et al. (2002) Hydrogen and synthesis gas by steam- and CO2 reforming. ChemInform, 47, pp. 65–139. doi.org/10.1002/chin.200317288.
- Kang, D., et al. (2019) Synthesis of Cu/Ni-La0.7 Sr0.3 Cr 0.5Mn 0.5O3–δ and its catalytic performance on dry methane reforming. Journal of Rare Earths, 37, pp. 585–593. doi.org/10.1016/j.jre.2018.10.016.
- Wang, F., et al. (2020) Low Temperature CO2 Reforming with Methane Reaction over CeO2 Modified Ni@SiO2 Catalysts. ACS Applied Materials Interfaces, 12, pp. 35022–35034. doi.org/10.1021/acsami.0c09371.
- Han, B., et al. (2020) Effect of calcination temperature on performance of Ni@SiO2 catalyst in methane dry reforming. Industrial & Engineering Chemistry Research, 59, pp. 13370–13379. doi.org/10.1021/acs.iecr.0c01213.
- Chein, R Y & Fung, W Y (2019) Syngas production via dry reforming of methane over CeO2 modified Ni/Al2O3 catalysts. International Journal of Hydrogen Energy, 44, pp. 14303–14315. doi.org/10.1016/j.ijhydene.2019.01.113.
- Ma, Q., et al. (2019) Combined methane dry reforming and methane partial oxidization for syngas production over high dispersion Ni based mesoporous catalyst. Fuel Processing Technology, 188, pp. 98–104.doi.org/10.1016/j.fuproc.2019.02.013.
- Abdullah, B., et al. (2017) Recent advances in dry reforming of methane over Ni-based catalysts. Journal of Cleaner Production, 162, pp. 170–185. doi.org/10.1016/j.jclepro.2017.05.176.
- Ali, S., et al. (2020) Ni-based nano-catalysts for the dry reforming of methane. Catalysis Today, 343, pp. 26–37. doi.org/10.1016/j.cattod.2019.04.066.
- Shen, J., et al. (2019) Ni/MgAl2O4 catalyst for low-temperature oxidative dry methane reforming with CO2. International Journal of Hydrogen Energy, 44, pp. 4616–4629. doi.org/10.1016/j.ijhydene.2019.01.027.
- Wanga, F., et al. (2018) CO2 reforming with methane over small-sized Ni@SiO2 catalysts with unique features of sintering-free and low carbon. Applied Catalysis B: Environmental, 235, pp. 26–35. doi.org/10.1016/j.apcatb.2018.04.069.
- Han, K., et al. (2021) Reducing carbon deposition and enhancing reaction stability by ceria for methane dry reforming over Ni@SiO2@CeO2 catalyst. Fuel, 291, p. 120182. doi.org/10.1016/j.fuel.2021.120182.
- Charisiou, N. D., et al. (2016) Syngas production via the biogas dry reforming reaction over nickel supported on modified with CeO2 and/or La2O3 alumina catalysts. Journal of Natural Gas Science and Engineering, 31, pp. 164–183. doi.org/10.1016/j.jngse.2016.02.021.
- Goula, M. A., et al. (2017) Syngas production via the biogas dry reforming reaction over Ni supported on zirconia modified with CeO2 or La2O3 catalysts. International Journal of Hydrogen Energy, 42, pp. 13724–13740. doi.org/10.1016/j.ijhydene.2016.11.196.
- Makri, M. M., et al. (2016) Effect of support composition on the origin and reactivity of carbon formed during dry reforming of methane over 5 wt% Ni/Ce 1−x MxO 2−δ (M= Zr 4+, Pr 3+) catalysts. Catalysis Today, 259, pp. 150–164. doi.org/10.1016/j.cattod.2015.06.010.
- Summa, P., et al. (2021) Co-Precipitated Ni-Mg-Al HydrotalciteDerived Catalyst Promoted with Vanadium for CO2 Methanation. Molecules, 26, p. 6506. doi.org/10.3390/molecules26216506.
- Swirka, K., et al. (2018) Dry reforming of methane over Zr- and Y-modified Ni/Mg/Al double-layered Hydroxides. Catalysis Communications, 117, pp. 26–32. doi.org/10.1016/j.catcom.2018.08.024.
- Swirk, K., et al. (2018) Yttrium promoted Ni-based double-layered hydroxides for dry methane Reforming. Journal of CO2 Utilization, 27, pp. 247–258. doi.org/10.1016/j.jcou.2018.08.004.
- Swirk, K., et al. (2019) Ce- and Y-Modified Double-Layered Hydroxides as Catalysts for Dry Reforming of Methane: On the Effect of Yttrium Promotion. Catalysts, 9, p. 56. doi.org/10.3390/catal9010056.
- Jacobson, A J (2010) Materials for Solid Oxide Fuel Cells. Chemistry of Materials, 22, pp. 660–674. doi.org/10.1021/cm902640j.
- Kim, W.Y., et al. (2019) Reduced perovskite LaNiO3 catalysts modified with Co and Mn for low coke formation in dry reforming of methane. Applied Catalysis A: General, 575, pp. 198–203. doi.org/10.1016/j.apcata.2019.02.029.
- Wei, T., et al. (2018) LaMnO3-based perovskite with in-situ exsolved Ni nanoparticles: A highly active, performance stable and coking resistant catalyst for CO2 dry reforming of CH4. Applied Catalysis A: General, 564, pp. 199–207. doi.org/10.1016/j.apcata.2018.07.031.
- Yang, Q., et al. (2018) Perovskite-type oxides as the catalyst precursors for preparing supported metallic nanocatalysts: A review. Industrial & Engineering Chemistry Research, 57, pp. 1–17. doi.org/10.1021/acs.iecr.7b03251.
- Bhattar, S., et al (2021) A review on dry reforming of methane over perovskite derived catalysts. Catalysis Today, 365, pp. 2–23. doi.org/10.1016/j.cattod.2020.10.041.
- Khalesi, A., et al. (2008) Production of Syngas by CO2 Reforming on MxLa 1−x Ni 0 .3Al 0.7 O 3−d (M = Li, Na, K). Industrial & Engineering Chemistry Research, 47, pp. 5892–5898. doi.org/10.1021/ie800111e.
- Pérez-Camacho, M. N., et al. (2014) Self-cleaning perovskite-type catalysts for the dry reforming of methane. Chinese Journal of Catalysis, pp. 1337–1346. doi.org/10.1016/S1872-2067(14)60187-X.
- Ruocco, C., et al. (2019) Methane dry reforming on Ru perovskites, AZrRuO3: Influence of preparation method and substitution of A cation with alkaline earth metals. Journal of CO2 Utilization, 30, pp. 222–231. doi.org/10.1016/j.jcou.2019.02.009.
- Wang, H., et al. (2009) Dry reforming of methane over bimetallic Ni-Co catalyst prepared from La(CoxNi1-x)0.5Fe 0.5 O 3 perovskite precursor: Catalytic activity and coking resistance. Applied Catalysis B: Environmental, 245, pp. 302–313. doi.org/10.1016/j.apcatb.2018.12.072.
- Wang, F., et al. (2016) Syngas production from CO2 reforming with methane over core-shell Ni@SiO2 catalysts. Journal of CO2 Utilization, 16, pp. 318–327. doi.org/10.1016/j.jcou.2016.09.001.
- Han, B., et al. (2020) Syngas production from methane steam reforming and dry reforming reactions over sintering-resistant Ni@SiO2 catalyst. Research on Chemical Intermediates, 46, pp. 1735–1748. doi.org/10.1007/s11164-019-04060-3.
- Dehghani, F., et al. (2020) Synthesis and Characterization of Mixed–Metal Oxide Nanoparticles (CeNiO3, CeZrO4, CeCaO3) and Application in Adsorption and Catalytic Oxidation–Decomposition of Asphaltenes with Different Chemical Structures. Petroleum Chemistry, 60, pp. 731–743. doi.org/10.1134/S0965544120070038.
- Harikrishnan, M. P., et al. (2020) Electrochemical performance of ANiO3 (A= La, Ce) perovskite oxide material and its device performance for supercapattery application. Electrochimica Acta, 362, p. 137095. doi.org/10.1016/j.electacta.2020.137095.
- Chen, J., et al. (2017) Visible-light-enhanced photothermocatalytic activity of ABO3-type perovskites for the decontamination of gaseous styrene. Applied Catalysis B: Environmental, 209, pp. 146–154. doi.org/10.1016/j.apcatb.2017.02.066
- Hu, Q., et al. (2020) Facile syntheses of cerium-based CeMO3 (M = Co, Ni, Cu) perovskite nanomaterials for high-performance supercapacitor electrodes. Journal of Materials Science, 55, pp. 8421–8434. doi.org/10.1007/s10853-020-04362-7.
- Garcı́a de la Cruz, R. M., et al. (2001) Role of bulk and surface structures of La1−x SrxNiO3 perovskite-type oxides in methane combustion. Applied Catalysis B: Environmental, 33, pp. 45–55. doi.org/10.1016/S0926-3373(01)00157-6.
- Wang, Y., et al. (2013) A simple co-nanocasting method to synthesize high surface area mesoporous LaCoO3 oxides for CO and NO oxidations. Microporous and Mesoporous Materials, 176, pp. 8–15. doi.org/10.1016/j.micromeso.2013.03.033
- Wei, Y., et al. (2015) Facile synthesis of three-dimensionally ordered macroporous LaFeO3- supported gold nanoparticle catalysts with high catalytic activity and stability for soot combustion. Catalysis Today, 245, pp. 37–45. doi.org/10.1016/j.cattod.2014.07.023.
- Sudhakaran, M.S.P., et al. (2019) Dry Reforming of Propane over γ-Al2O3 and Nickel Foam Supported Novel SrNiO3 Perovskite Catalyst. Catalyst, 9, p. 68. doi.org/10.3390/catal9010068.
- . Pino, L., et al. (2020) Kinetic study of the methane dry (CO2) reforming reaction over the Ce 0.70 La 0.20 Ni 0.10 O2−δ catalyst. Catalysis Science & Technology, 10, pp. 2652–2662. doi.org/10.1039/C9CY02192B.
- Ostrovskii, N M (2005) Problems in the Study of Catalyst Deactivation Kinetics. Kinetics and Catalysis, 46, pp. 693–704. doi.org/10.1007/s10975-005-0124-6.
- Moral, A., et al. (2018) Syngas production by means of biogas catalytic partial oxidation and dry reforming using Rh-based catalysts. Catalysis Today. 299, pp. 280–288 doi.org/10.1016/j.cattod.2017.03.049.
- Verykios, X., et al. (2003). Mechanistic aspects of the reaction of CO2 reforming of methane over Rh/Al2O3 catalyst. Applied Catalysis A: General, 255, pp. 101–111 doi.org/10.1016/S0926-860X(03)00648-3.
- Choudhary, V. R., et al. (1996) Oxidative Conversion of Methane to Syngas over LaNiO3 Perovskite with or without Simultaneous Steam and CO2 Reforming Reactions: Influence of Partial Substitution of La and Ni. Journal of Catalysis, 163, pp. 312–318. doi.org/10.1006/jcat.1996.0332.
- Lima, S. M., et al. (2006) Structural features of La1−xCexNiO3 mixed oxides and performance for the dry reforming of methane. Applied Catalysis A: General, 311, pp. 94–104. doi.org/10.1016/j.apcata.2006.06.010.
- Valderrama, G., et al. (2005) Dry reforming of methane over Ni perovskite type oxides. Catalysis Today, 107–108, pp. 785–791. doi.org/10.1016/j.cattod.2005.07.010.
- Das, S., et al. (2020) Effect of Partial Fe Substitution in La 0.9 Sr 0.1NiO3 Perovskitederived Catalysts on Reaction Mechanism of Methane Dry Reforming. ACS Catalysis, 10, pp. 12466–12486. doi.org/10.1021/acscatal.0c01229.
- Komarala, E. P., et al. (2020) Effect of Fe and Mn Substitution in LaNiO3 on Exsolution, Activity, and Stability for Methane Dry Reforming. Catalysts, 10, p. 27. doi.org/10.3390/catal10010027.
- Mousavi, M., et al. (2020) Dry Reforming of Methane by La0.5 Sr 0.5 NiO3 Perovskite Oxides: Influence of Preparation Method on Performance and Structural Features of the Catalysts. Journal of Chemical Technology & Biotechnology, 95, pp. 2911–2920. doi.org/10.1002/jctb.6451.
- Voorhoeve, R J H (1977) 5—Perovskite-Related Oxides as Oxidation—Reduction Catalysts. In Advanced Materials in Catalysis; Burton, J. J., Garten, R. L., Eds.; Academic Press: New York, NY, USA, pp. 129–180.
- Messaoudi, H., et al. (2018) Study of LaxNiOy and LaxNiOy/MgAl2O4 catalysts in dry reforming of methane. Journal of CO2 Utilization, 24, pp. 40–49. doi.org/10.1016/j.jcou.2017.12.002.