Nanoparticles, particularly protein-derived inorganic nanoparticles, are in great demand. Proteins provide a reducing and stabilizing environment during nanoparticle synthesis. Research published in the International Journal of Nanomedicine discusses the synthesis of AgNPs with Benincasa hispida pulp protein extract before exploring its characterization and evaluation of its biological activity.
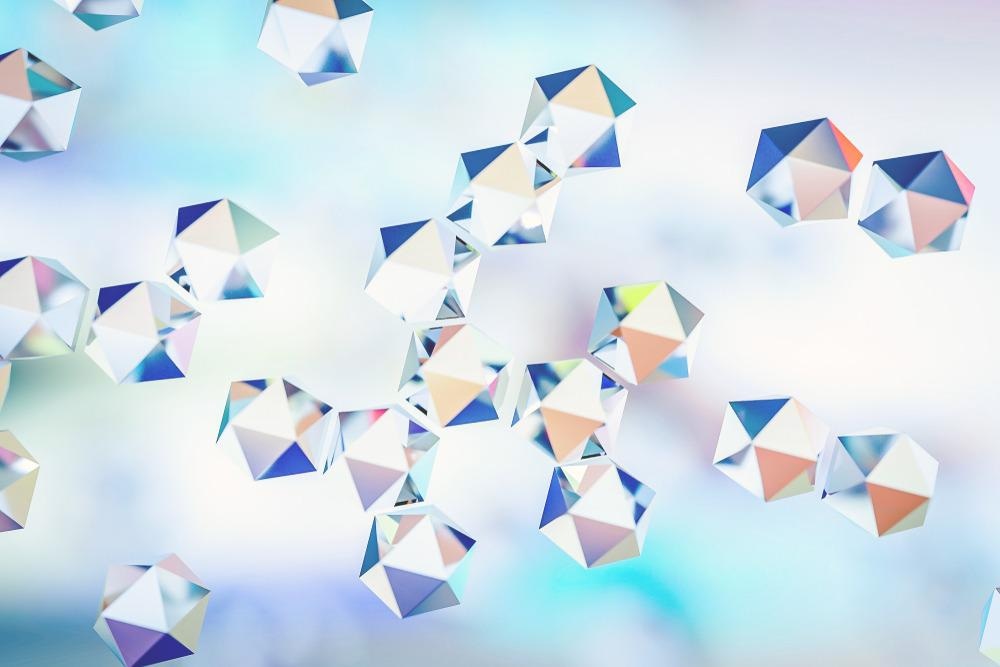
Image Credit: CI Photos/Shutterstock.com
Recently, the popularity of inorganic nanomaterials has seen a vast increase. This demand is due to their numerous applications in various macroeconomic industries.
Silver nanoparticles (AgNPs) are used in different fields, specifically as a broad-spectrum antimicrobial agent. Their use also extends to many commercial consumer products like cosmetics, wound dressings, antimicrobial socks, and personal care items. With most nanoparticles, both physical and chemical methods are employed for their synthesis. Each has its own set of disadvantages, with biocompatibility tending to top the list.
Green synthesis, synthesizing nanoparticles from organic products like bacteria, fungi and plants, is a promising area of nanoparticle research. The phytochemicals from plants can stabilize and reduce agents in synthesizing metal nanoparticles.
Benincasa (Cucurbitaceae), a monotypic genus, has a single species named Benincasa hispida. The fruits of B. hispida are commonly used as a diuretic, and the seeds have been shown to have antiangiogenic effects on prostate cells. The mature fruits of B. hispida harbor proteins, dietary fibers, sterols, enzymes, flavonoid C-glycoside, sugars, phenolic acids, and trace minerals.
In this study, researchers devised a route to synthesize AgNPs with B. hispida pulp protein extract, contributing to the field of green nanotechnology. The generated nanoparticles were found to be highly biocompatible and stable.
Methodology
Extraction of B. hispida fruit protein took place by finely grinding cleaned fruits and later homogenizing the powdered samples. The proteins were then extracted, and the exact amount was calculated. The extracted protein was then centrifuged, the purified protein extract was utilized for synthesizing AgNPs. The protein concentration was determined with Bradford assay and the purified proteins were examined on 7.5% SDS-PAGE.
The silver nanoparticles were then synthesized from the B. hispida fruit proteins of varying concentrations. Silver nitrate salt was added at different temperatures to obtain the desired-sized nanoparticles.
The synthesized nanoparticles were later characterized with UV-Visible Spectroscopy, the optical density was measured, and the average particle size of AgNPs was also evaluated.
Transmission Electron Microscopy (TEM) was used to investigate the size and the zeta-potential was evaluated with a Zetasizer Nano-ZS. Fourier-Transform Infrared Spectroscopy (FTIR) was used to reveal the information on protein–nanoparticle associations.
The antibacterial activity of the synthesized AgNPs was evaluated with the agar well diffusion technique against E. coli, S. aureus, S. enteric, and S. epidermis. From here, the minimum inhibition concentration of AgNPs was determined along with the anti-biofilm potential of AgNPs.
The team also investigated the anticancer potential of AgNPs against A549 and NRK cells, and studied the cytomorphological changes of the treated A549 cells and the variations in the nuclear morphology of cells.
Results and Discussion
The native charge of proteins performs a vital role in nanoemulsion stability (see Figure 1).
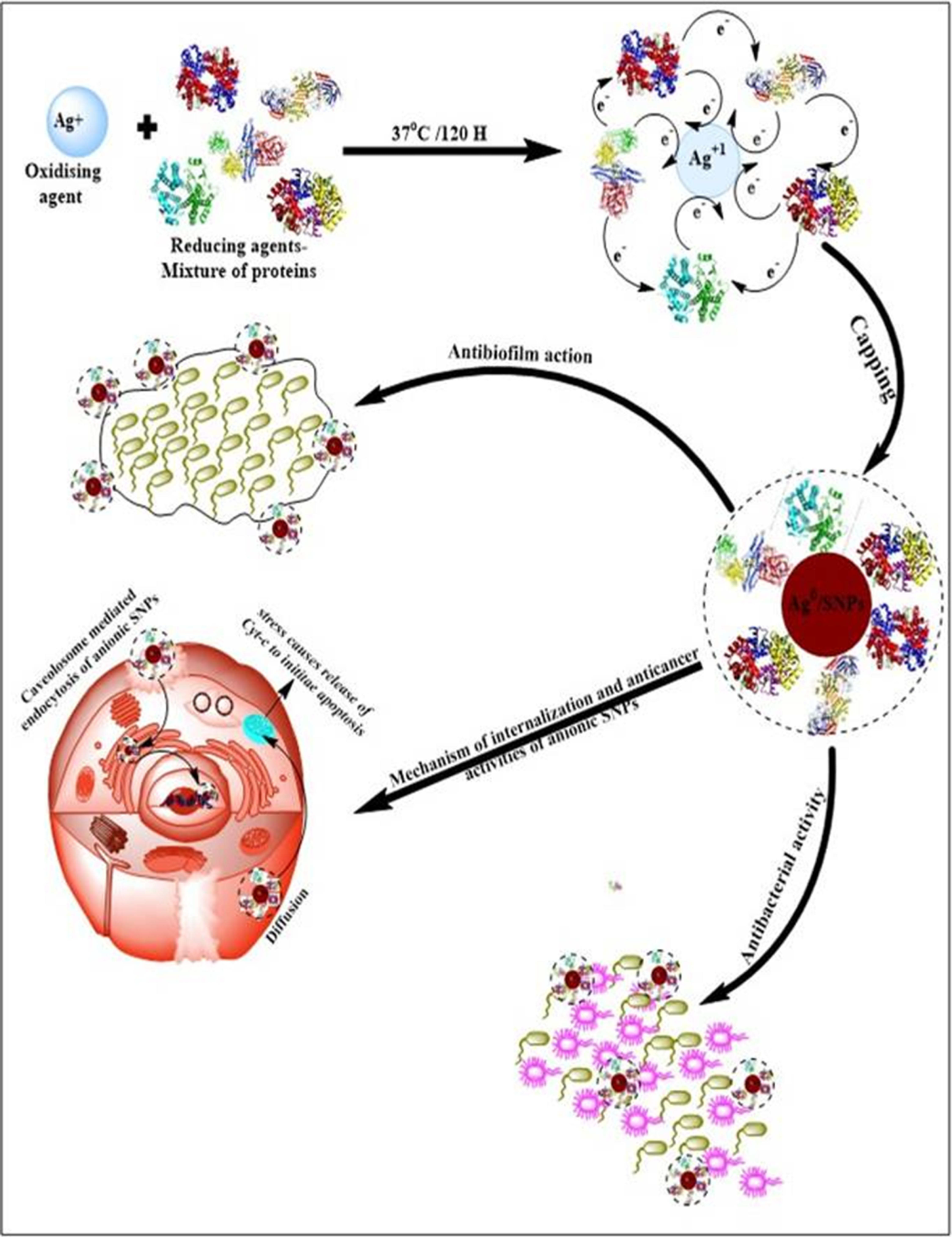
Figure 1. Schematic representation of the mechanism of B. hispida fruit proteins mediated synthesis of biogenic AgNPs and their roles in anticancer, antibacterial, and antibiofilm agents. Image Credit: Baker, et al., 2021
Figure 2 reveals the characterization of the prepared AgNPs using different techniques.
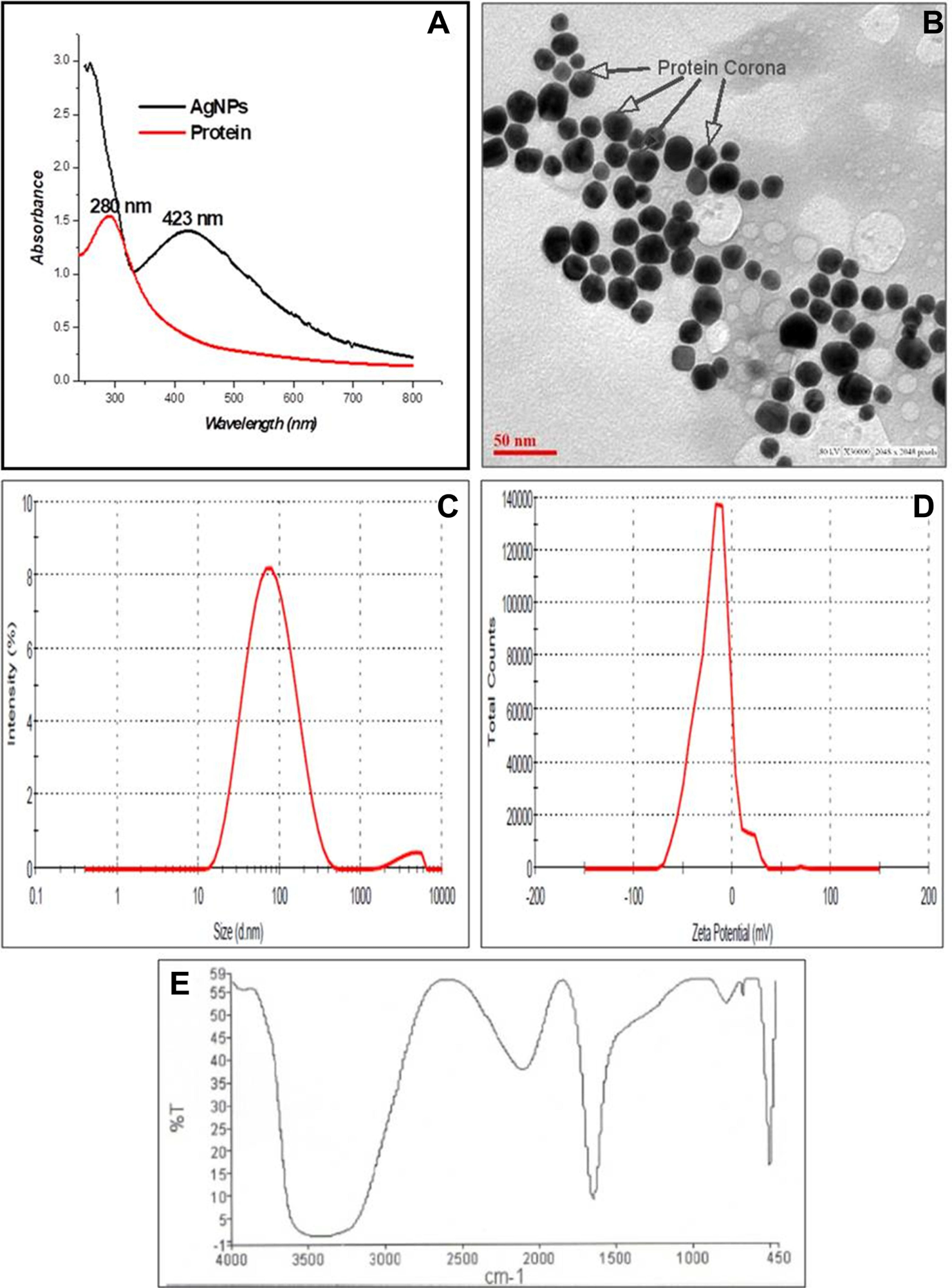
Figure 2. Characterization of B. hispida fruit proteins mediated synthesis of AgNPs by physical techniques: (A) UV-visible spectrum, (B) TEM Micrograph with light gray protein Corona mark by an arrow, (C) size distribution by DLS (D) zeta potential (E) surface characterization by FTIR spectrum. Image Credit: Baker, et al., 2021
The average size was found to be 27 ± 1 nm, and further analysis revealed the shape to be almost spherical. The zeta potential value for AgNPs was –19.7 ± 0.2 mV. FT analysis showed peaks that are characteristics of C=O amide groups of the amide I linkage.
The antibacterial activity of AgNPs examined against the four types of bacteria (see Figure 3) showed broad-spectrum activity.
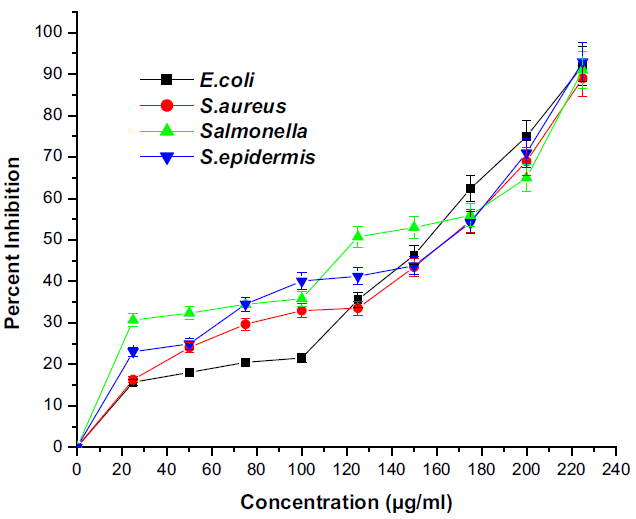
Figure 3. Graph showing the antibacterial potential of silver nanoparticles. All the data were expressed in the mean ± SD of three experiments. Image Credit: Baker, et al., 2021
Antibacterial activity can be due to several mechanisms like ROS generation, enhanced permeability, change in membrane fluidity, and membrane integrity loss, interrupting the actin, binding to the 30S subunit of ribosomes.
The biosynthesized AgNPs can obstruct the biofilm formation of different pathogenic bacteria; a significant reduction of 70-90% was seen in the test conducted (see Figure 4). This antibiofilm formation was dose-dependent and that the increased concentration of AgNPs decreased the biofilm formation.
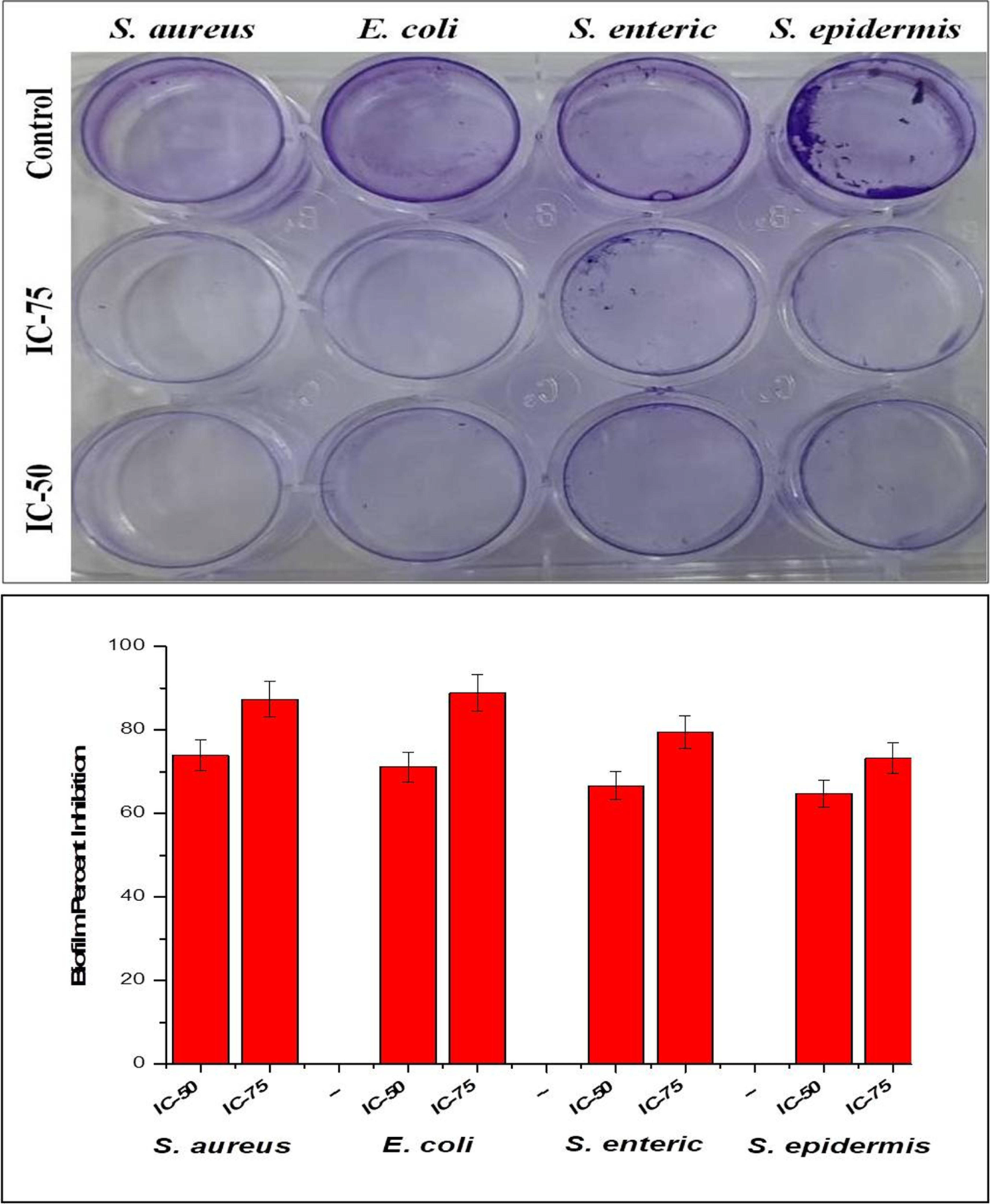
Figure 4. Image and graph showing inhibition of biofilm formation of silver nanoparticles. All the data were expressed in the mean ± SD of three experiments. Image Credit: Baker, et al., 2021
Anticancer studies revealed that the AgNPs were active against A549 cells (see Figure 5A), however, they did not exhibit any activity against NRK cells up to 200 µM (see Figure 5B).
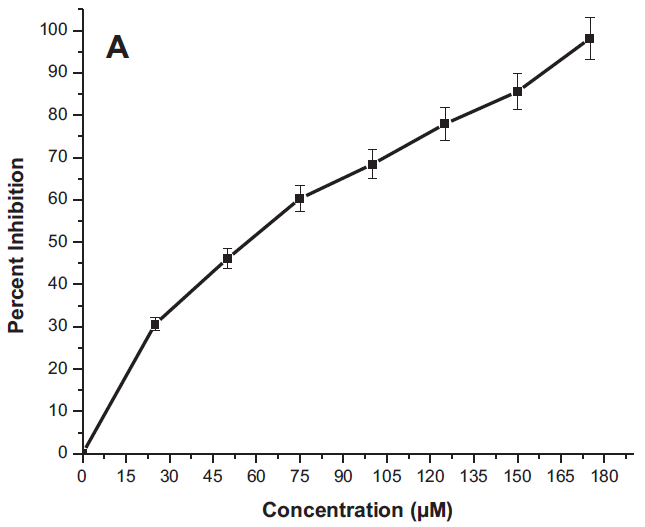
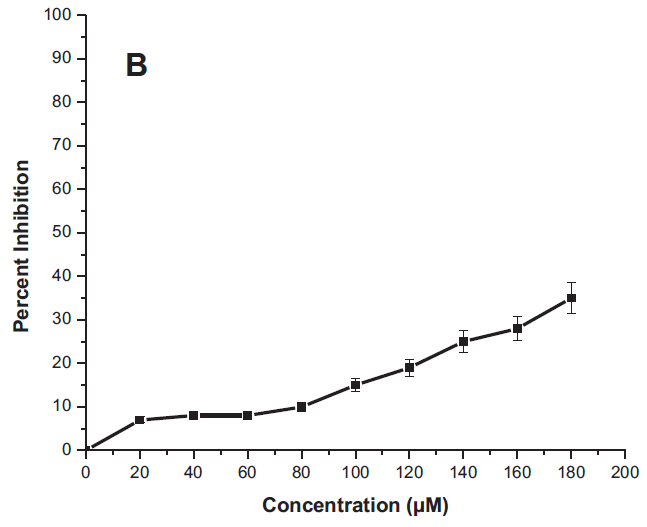
Figure 5. The cytotoxicity study graph of MTT assay for AgNPs treatment against (A) A549 cells and (B) NRK cells. Image Credit: Baker, et al., 2021
Also, the anticancer effect is dose-dependent, the activity increases with an increase in the concentration of AgNPs. Figure 6 indicates the morphological changes of A549 cells after incubation with various concentrations (IC25, IC50 & IC75) of AgNPs.
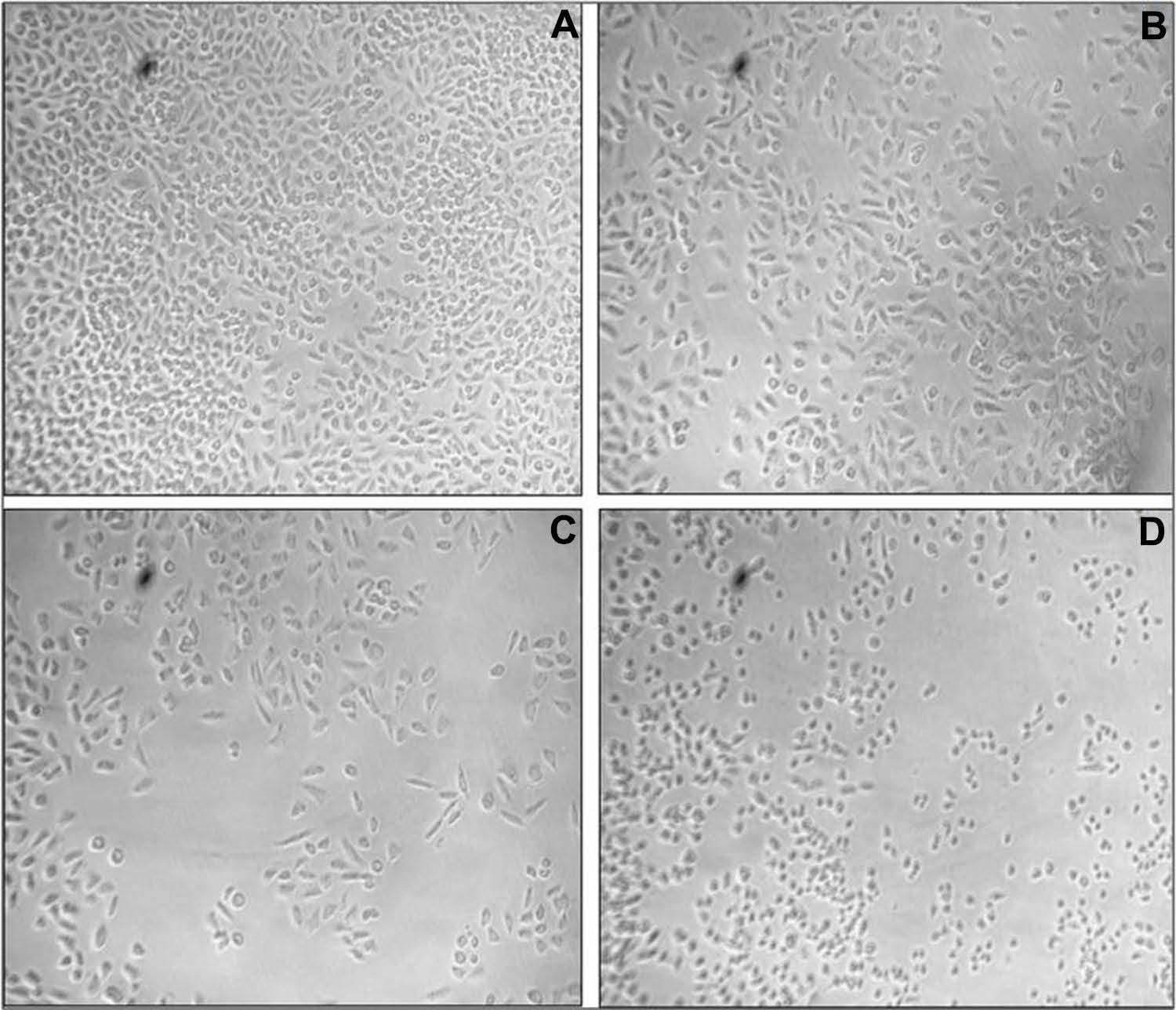
Figure 6. The cytomorphological study of AgNPs treated A549 cells (A) control, (B) IC25 (C) IC50, (D) IC75 at 20× magnification. Image Credit: Baker, et al., 2021
Observations show cells having deformed morphologies while some cells had their plasma membrane intact, indicating the start of apoptosis.
The AgNPs' interaction with nuclear materials was analyzed using fluorescent dye. The treated cells showed apoptotic impact (see Figure 7A and B) when compared with untreated cells. It was noted that AgNPs produced more fluorescence compared to untreated cells (Figure 8A).
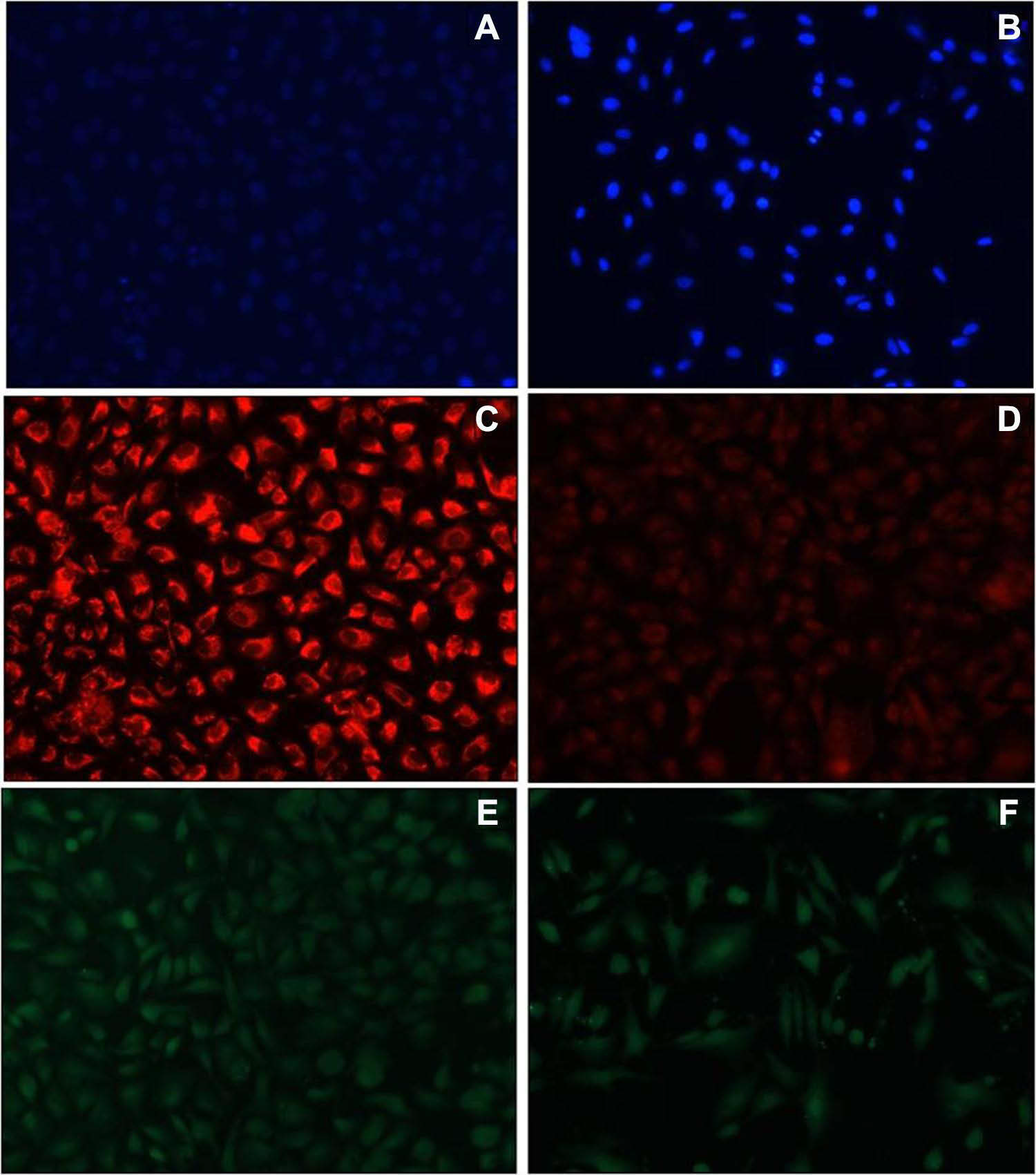
Figure 7. Images observed for AgNPs treated cells under phase contrast microscope after 48 hours of treatment for DAPI staining (A) control (B) treated cells; for Mito Tracker Red staining (C) control (D) treated cells and for DCFDA staining (E) control (F) treated cells. Image Credit: Baker, et al., 2021
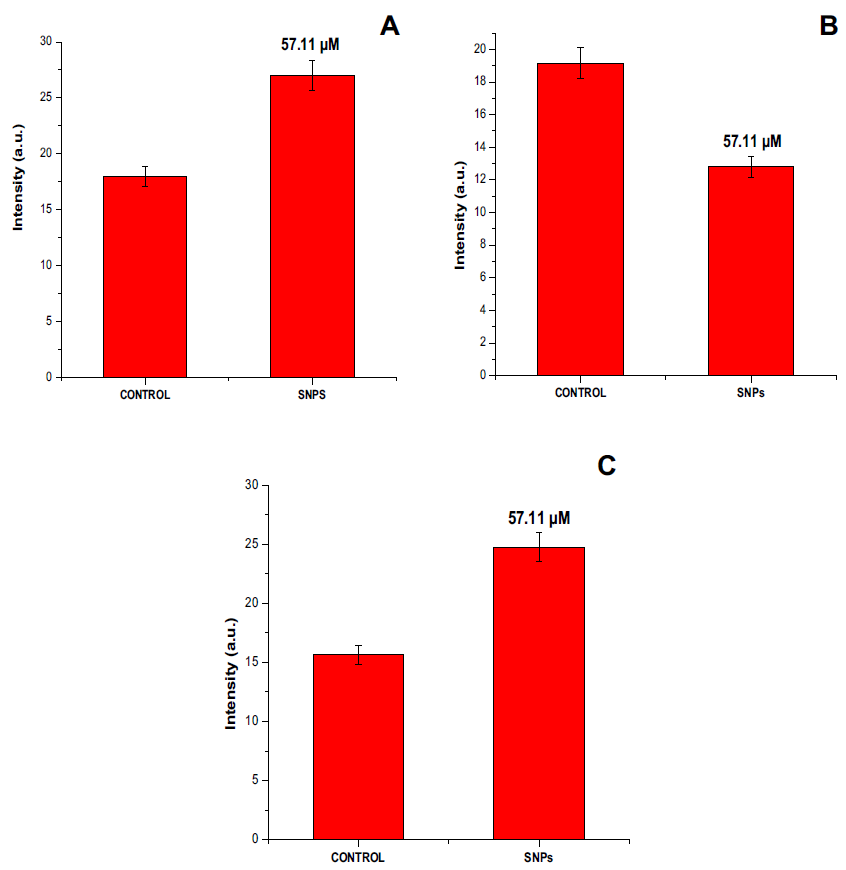
Figure 8. Graphical representation in terms of percent (A) nuclear condensation, (B) mitochondrial content, and (C) intracellular ROS generation. All the data were expressed in the mean ± SD of three experiments. Image Credit: Baker, et al., 2021
It was seen that AgNPs can disrupt the mitochondrial membrane potential (ΔΨm) of A549 cells. ROS generation of A549 cells after interaction with AgNPs was evaluated and the intensity of fluorescence was a direct measure of ROS generated in the cells. A greater intensity of fluorescence was observed while the untreated cells showed no fluorescence.
Conclusion
Researchers detailed a green protocol for producing silver nanoparticles with the fruit protein extract of Benincasa hispida. The synthesized silver nanoparticles enhanced the potential of Benincasa hispida fruit protein, and showed efficient antibacterial, antibiofilm, and anticancer activities. However further studies are required to analyze their toxicity.
Journal Reference
Baker, A., Iram, S., Syed, A., Elgorban, A. M., Bahkali, A. H., Ahmad, K., Khan, M. S., Kim, J. (2021) Fruit Derived Potentially Bioactive Bioengineered Silver Nanoparticles. International Journal of Nanomedicine, 16, pp. 7711–7726. Available online: https://www.dovepress.com/getfile.php?fileID=75944.
References and Further Reading
- Ovais, M., et al. (2018) Biosynthesis of Metal Nanoparticles via Microbial Enzymes: A Mechanistic Approach. International Journal of Molecular Sciences, 19 (12), p. 4100. doi.org/10.3390/ijms19124100.
- Ahmad, S. A., et al. (2020) Bactericidal activity of silver nanoparticles: a mechanistic review. Materials Science for Energy Technologies, 3, pp. 756–759. doi.org/10.1016/j.mset.2020.09.002.
- Sim, W., et al. (2018) Antimicrobial silver in medicinal and consumer applications: a patent review of the past decade (2007–2017). Antibiotics, 7(4), p. 93. doi.org/10.1016/j.mset.2020.09.002.
- Deore, S. L., et al. (2009) In vitro antioxidant activity and quantitative estimation of phenolic content of Lagenaria siceraria. Rasayan Journal of Chemistry.
- Pinzaru, I., et al. (2018) Stable PEG-coated silver nanoparticles–A comprehensive toxicological profile. Food and Chemical Toxicology, 111, pp.546–556. doi.org/10.1016/j.fct.2017.11.051.
- Guzmán, M. G., et al. (2009) Synthesis of silver nanoparticles by chemical reduction method and their antibacterial activity. International Journal of Chemical and Biomolecular Engineering, 2(3), pp. 104–111.
- Van Dong, P., et al. (2012) Chemical synthesis and antibacterial activity of novel-shaped silver nanoparticles. International Nano Letters, 2(1), pp. 1–9. doi.org/10.1186/2228-5326-2-9.
- Tortella, G. R., et al. (2020) Silver nanoparticles: toxicity in model organisms as an overview of its hazard for human health and the environment. Journal of Hazardous Materials, 390, p. 121974. doi.org/10.1016/j.jhazmat.2019.121974.
- Elbeshehy, E. K. F., et al. (2015) Silver nanoparticles synthesis mediated by new isolates of Bacillus spp., nanoparticle characterization and their activity against Bean Yellow Mosaic Virus and human pathogens. Frontiers in Microbiology, 6, p. 453. doi.org/10.3389/fmicb.2015.00453.
- Gao, Y., et al. (2020) Potentials of nanotechnology in treatment of methicillin-resistant Staphylococcus aureus. European Journal of Medicinal Chemistry, p. 113056. doi.org/10.1016/j.ejmech.2020.113056.
- Zhao, Y., et al. (2021) Nanomaterial-based strategies in antimicrobial applications: progress and perspectives. Nano Research, 14, pp. 1–25. doi.org/10.1007/s12274-021-3417-4.
- Chen, Y., et al. (2020) Nanomaterials-based photothermal therapy and its potentials in antibacterial treatment. Journal of Controlled Release, 328, pp. 251–262. doi.org/10.1007/s12274-021-3417-4.
- Yu, C.-H., et al. (2020) Understanding the sheet size-antibacterial activity relationship of graphene oxide and the nano-bio interaction-based physical mechanisms. Colloids and Surfaces B Biointerfaces, 191, p. 111009. doi.org/10.1016/j.colsurfb.2020.111009.
- Phull, A.-R., et al. (2020) Synthesis of silver nanoparticles using euphorbia wallichii extract and assessment of their bio-functionalities. Medicinal chemistry (Los Angeles), 16 (4), pp. 495–506. doi.org/10.2174/1573406415666191111143213.
- Ganguly, S., et al. (2018) Natural saponin stabilized nano-catalyst as efficient dye-degradation catalyst. Nano-Structures & Nano-Objects, 16, pp. 86–95. doi.org/10.1016/j.nanoso.2018.05.002.
- Khan, S., et al. (2015) A novel process for size controlled biosynthesis of gold nanoparticles using bromelain. Material Letters, 159, pp. 373–376. doi.org/10.1016/j.matlet.2015.06.118.
- . Khan, S., et al. (2014) Approach for the synthesis of gold nanoparticles using trypsin. Journal of Computational and Theoretical Nanoscience, 20(5–6), pp. 1061–1065. doi.org/10.1166/asl.2014.5481.
- Xu, X & Man, L (2021) Papain mediated synthesized gold nanoparticles encore the potency of bioconjugated Flutamide. Current Pharmaceutical Biotechnology, 22(4), pp. 557–568. doi.org/10.2174/1389201021666200227121144.
- Jayasree, T., et al. (2011) Diuretic effect of chloroform extract of Benincasa hispida rind (pericarp) in Sprague-Dawley rats. International Journal of Applied Biology and Pharmaceutical Technology, 22, pp. 94–99.
- Dong, M. Y., et al. (1995) Study of Benincasa hispida contents effective for protection of kidney. The Journal of Agricultural Science, 11, pp. 46–52.
- Shih, C.-Y.T., et al. (2001) Purification of an osmotin-like protein from the seeds of Benincasa hispida and cloning of the gene encoding this protein. Plant Science, 160(5), pp. 817–826. doi.org/10.1016/S0168-9452(00)00450-7.
- Atiwetin, P., et al. (2006) Serine proteinase inhibitor from wax gourd (Benincasa hispida [Thunb] Cogn.) seeds. Bioscience, Biotechnology, and Biochemistry, 70(3), pp. 743–745. doi.org/10.1271/bbb.70.743.
- Zaini, N. A. M., et al. (2011) Kundur [Benincasa hispida (Thunb.) Cogn.]: a potential source for valuable nutrients and functional foods. Food Research International, 44(7), pp. 2368–2376. doi.org/10.1016/j.foodres.2010.10.024.
- Qadrie, Z. L., et al. (2009) Antinociceptive and anti-pyretic activity of Benincasa hispida (Thunb.) cogn. in Wistar albino rats. Pakistan Journal of Pharmaceutical Sciences. 22(3), p. 45.
- Shakya, A., et al. (2019) Assessment of neurobehavioral properties of hydroalcoholic extract of Benincasa hispida (Thunb.) Cogn. Fruit Pulp in Mice. Journal of Biologically Active Products from Nature, 9(4), pp. 299–310. doi.org/10.1080/22311866.2019.1684367.
- Sabale, V., et al. (2011) Formulation and in vitro evaluation of the topical antiageing preparation of the fruit of Benincasa hispida. Journal of Ayurveda and Integrative Medicine, 2(3), p. 124. doi.org/10.1080/22311866.2019.1684367.
- Jiang, X., et al. (2016) Prediction of the antiglycation activity of polysaccharides from Benincasa hispida using a response surface methodology. Carbohydrate Polymers, 151, pp. 358–363. doi.org/10.1080/22311866.2019.1684367.
- Nadhiya, K., et al. (2016) Antiobesity effect of Benincasa hispida Fruit extract in high fat diet fed Wistar albino rats. International Journal of Pharmacology and Clinical Research, 8(12), pp. 1590–1599. doi.org/10.1080/22311866.2019.1684367.
- Gill, N. S., et al. (2011) Evaluation of free radical scavenging and antiulcer potential of methanolic extract of Benincasa hispida seeds. Research Journal of Medicinal Plant, 5(5), pp. 596–604. doi.org/10.1080/22311866.2019.1684367.
- Yenda, B., et al. (2014) In vitro antioxidant activity studies on leaves of Benincasa hispida (Thunb.) Cogn. Research Journal of Pharmaceutical, Biological and Chemical Sciences, 5, pp. 141–147. doi.org/10.1080/22311866.2019.1684367.
- Huang, H., et al. (2004) Antioxidant and angiotension-converting enzyme inhibition capacities of various parts of Benincasa hispida (wax gourd). Food/Nahrung, 48(3), pp. 230–233. doi.org/10.1002/food.200300428.
- Varghese, H. S., et al. (2013) Nephroprotective activity of Benincasa hispida (Thunb.) Cogn. Fruit extract against paracetamol induced nephrotoxicity in rats. Journal of Pharmaceutical, Biological and Chemical Sciences, 4(1), pp. 322–332.
- Lee, K.-H., et al. (2005) Anti-angiogenic effect of the seed extract of Benincasa hispida Cogniaux. Journal of Ethnopharmacology, 97(3), pp. 509–513. doi.org/10.1016/j.jep.2004.12.008.
- Natarajan, D., et al. (2003) Antimicrobial studies on methanol extract of Benincasa hispida cogn., fruit. Ancient Science of Life, 22(3), p. 98.
- Moon, M. K., et al. (2009) Effect of Benincasa hispida Cogniaux on high glucose-induced vascular inflammation of human umbilical vein endothelial cells. Vascular Pharmacology, 50(3–4), pp.116–122. doi.org/10.1016/j.vph.2008.11.007.
- Wingfield, P T (2016) Protein precipitation using ammonium sulfate. Current Protocols in Protein Science, 84(1), A–3F. doi.org/10.1002%2F0471140864.psa03fs13.
- Khan, S., et al. (2018) Biogenic pentagonal silver nanoparticles for safer and more effective antibacterial therapeutics. International Journal of Nanomedicine, 13, p. 7789. doi.org/10.2147/IJN.S168224.
- Iram, S., et al. (2019) Cisplatin bioconjugated enzymatic GNPs amplify the effect of cisplatin with acquiescence. Scientific Reports, 9(1), pp. 1–16. doi.org/10.1038/s41598-019-50215-y.
- Khan, M. S., et al. (2008) Antibacterial activity of synthesized 2, 4, 5-trisubstituted imidazole derivatives. Chemical Biology & Drug Design, 72(3), pp. 197–204. doi.org/10.1038/s41598-019-50215-y.
- Baker, A., et al. (2020) Sericin-functionalized GNPs potentiate the synergistic effect of levofloxacin and balofloxacin against MDR bacteria. Microbial Pathogenesis, 148, p. 104467. doi.org/10.1016/j.micpath.2020.104467.
- Kalishwaralal, K., et al. (2010) Silver nanoparticles impede the biofilm formation by Pseudomonas aeruginosa and Staphylococcus epidermidis. Colloids and Surfaces B: Biointerfaces, 79(2), pp. 340–344. doi.org/10.1016/j.colsurfb.2010.04.014.
- Baker, A., et al. (2021) Silk cocoon-derived protein bioinspired gold nanoparticles as a formidable anticancer agent. Journal of Biomedical Nanotechnology, 17(4), pp. 615–626. doi.org/10.1016/j.micpath.2020.104467.
- Leite, F. L., et al. (2012) Theoretical models for surface forces and adhesion and their measurement using atomic force microscopy. International Journal of Molecular Sciences, 13(10), pp. 12773–12856. doi.org/10.3390/ijms131012773.
- Huang, J., et al. (2007) Biosynthesis of silver and gold nanoparticles by novel sundried Cinnamomum camphora leaf. Nanotechnology, 18(10), p. 105104.
- Soliman, W. E., et al. (2020) Therapeutic applications of biostable silver nanoparticles synthesized using peel extract of Benincasa hispida: antibacterial and anticancer activities, Nanomaterials, 10(10), p. 1954. doi.org/10.3390/nano10101954.
- Krutyakov, Y. A., et al. (2008) Synthesis and properties of silver nanoparticles: advances and prospects. Russian Chemical Reviews, 77(3), p. 233. doi.org/10.3390/ijms131012773.
- Jena, P., et al. (2020) Bimetallic gold–silver nanoparticles mediate bacterial killing by disrupting the actin cytoskeleton MreB. Nanoscale, 12(6), pp. 3731–3749. doi.org/10.1039/C9NR10700B.
- Rai, M. K., et al. (2012) Silver nanoparticles: the powerful nanoweapon against multidrug-resistant bacteria. Journal of Applied Microbiology, 112(5), pp. 841–852 doi.org/10.1111/j.1365-2672.2012.05253.x.
- Liao, S., et al. (2019) Antibacterial activity and mechanism of silver nanoparticles against multidrug-resistant Pseudomonas aeruginosa. International Journal of Nanomedicine, 14, p. 1469. doi.org/10.2147/ijn.s191340.
- Shrivastava, S., et al. (2007) Characterization of enhanced antibacterial effects of novel silver nanoparticles. Nanotechnology, 18(22), p. 225103. doi.org/10.1088/0957-4484/18/22/225103.
- Mirzajani, F., et al. (2011) Antibacterial effect of silver nanoparticles on Staphylococcus aureus. Research in Microbiology, 162 (5), pp. 542–549. doi.org/10.1016/j.resmic.2011.04.009.
- Joo, H.-S., et al. (2012) Molecular basis of in vivo biofilm formation by bacterial pathogens. Chemical Biology, 19(12), pp. 1503–1513. doi.org/10.1016/j.chembiol.2012.10.022.
- Park, H.-J., et al. (2013) Removal characteristics of engineered nanoparticles by activated sludge. Chemosphere, 92(5), pp. 524–528. doi.org/10.1016/j.chemosphere.2013.03.020.
- Ehrlich, G D & Arciola C (2012) From Koch’s postulates to biofilm theory the lesson of bill costerton. International Journal of Artificial Organs, 35, pp. 695–699. doi.org/10.5301/ijao.5000169.
- Maira-Litrán, T., et al. (2012) Synthesis and evaluation of a conjugate vaccine composed of Staphylococcus aureus poly-N-acetylglucosamine and clumping factor A. PLoS ONE, 7(9), p. e43813. doi.org/10.1371/journal.pone.0043813.
- Al-Sheddi, E. S., et al. (2018) Anticancer potential of green synthesized silver nanoparticles using extract of nepeta deflersiana against human cervical cancer cells (HeLA). Bioinorganic Chemistry and Applications, pp. 1–12. doi.org/10.1155/2018/9390784.
- Gurunathan, S., et al. (2009) Antiangiogenic properties of silver nanoparticles. Biomaterials, 30(31), pp. 6341–6350. doi.org/10.1016/j.biomaterials.2009.08.008.
- Mollick, M. M. R., et al. (2019) Studies on green synthesized silver nanoparticles using Abelmoschus esculentus (L.) pulp extract having anticancer (in vitro) and antimicrobial applications. Arabian Journal of Chemistry, 12 (8), pp. 2572–2584. doi.org/10.1016/j.arabjc.2015.04.033.
- Chithrani, B. D., et al. (2006) Determining the size and shape dependence of gold nanoparticle uptake into mammalian cells. Nano Letters, 6(4), pp. 662–668. doi.org/10.1021/nl052396o.
- Valko, M., et al. (2007) Free radicals and antioxidants in normal physiological functions and human disease. The International Journal of Biochemistry & Cell Biology, 39(1), pp. 44–84. doi.org/10.1016/j.biocel.2006.07.001.
- Yan, L., et al. (2013) Chemical mechanisms of the toxicological properties of nanomaterials: generation of intracellular reactive oxygen species. Chemistry: An Asian Journal, 8(10), pp. 2342–2353. doi.org/10.1002/asia.201300542.
- Sharma, P., et al. (2012) Reactive oxygen species, oxidative damage, and antioxidative defense mechanism in plants under stressful conditions. Journal of Botany, 2012, p. 55. doi.org/10.1155/2012/217037.