Engineered nanomaterials (ENMs) and nanoparticles (NPs) are materials whose sizes range between 1 and 100 nm. These materials have many desirable chemical, biological, physical, electrical, magnetic, optical, and other properties. The application of ENMs and NPs has been increasing continuously in various fields, such as medicine, electronics, materials, energy, and biotechnology.
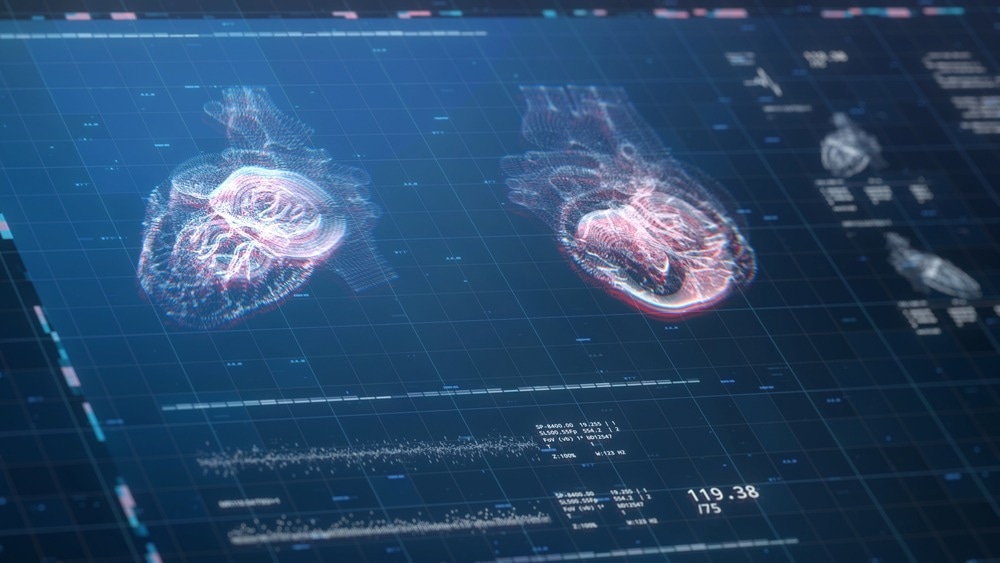
Image Credit: SquareMotion/Shutterstock.com
Why is it Important to Detect NPs and ENMs in Biological Systems?
NPs are commonly found in many common everyday products, such as cosmetics, clothes, and food preservatives. There is a high possibility that every individual is unknowingly exposed to various NPs. As a result, recently, there has been an increased study on nanotoxicology. It is important to understand how NPs and engineered nanomaterials (ENMs) impact humans and the environment. Advanced analytical tools, such as Magnetic Resonance Imaging (MRI), have been used for this purpose.
Compared to the larger particles or bulk materials, the small size of NPs provides them with differentiating characteristics, which allows diffusivity across cell membranes. The surface charge of ENMs has been associated with unique cell interactions, such that anionic and neutral ENMs offer lower toxicity than cationic materials.
It has been noted that the surface charge of ENMs influences the overall shape of the particles, which in turn can modify cell membranes and affect the cellular uptake mechanism. Surface coatings of ENMs can alter toxicity by delivering an excess of electrostatic forces, atomic layer deposition, and molecular adhesion, causing cell death.
The elemental composition of ENMs also affects the overall toxicity in biological systems. Nanotoxicity remains an under-researched area, primarily due to limitations in analytical instrumentation and assays that fail to measure ENMs in the environmental and biological matrices.
As stated above, NPs and ENMs are introduced in the biological system primarily through consumer products. These are introduced to humans via inhalation, skin absorption, and ingestion. ENMs can affect the mitochondrial function of cells and release reactive oxidative species (ROS).
A high ROS level can lead to oxidative damage to healthy cells and impair cellular metabolism. ROS accumulation transforms healthy cells into cancerous ones. Introducing NPs in a biological system can promote ROS generation via multiple pathways based on the type of NPs.
Working Principle of Non-Invasive Magnetic Resonance Imaging (MRI)
MRI is a commonly used non-invasive analysis that offers detailed images of a patient’s soft tissues. When a magnetic field (B0) is introduced to the hydrogen protons in the body, which were previously randomly distributed, they get aligned accordingly. Most protons, also designated as spins, point toward the direction of the magnetic field and are known as low-energy spins. In contrast, a small quantity of energetic protons point in the magnetic field's opposite direction and are known to be high-energy spins.
MRI captures the magnetic behavior of millions of protons. Instead of analyzing each spin individually, MRI captures the averaged sum of groups of protons, which is known as net magnetization (M). When a magnetic field is applied and the vector M points towards the direction of the magnetic field reaching an equilibrium, it is known as equilibrium magnetization M0. Subsequently, a radiofrequency pulse is incorporated that disrupts M0, forcing it to spin out of equilibrium.
When a radiofrequency pulse is paused, M returns to the equilibrium state via the relaxation phenomenon. The time required for M to return to the equilibrium state and electromagnetic energy released during the process is measured. This information is converted into detailed images on a computer, which can be visualized by clinicians.
There are two relaxation processes, namely, longitudinal or spin-lattice relaxation and transverse or spin-spin relaxation. Some regions of the body generate brighter signals compared to the surroundings. These regions are known as positive contrast and can be easily identified. However, certain regions produce darker signals following their surroundings and have to be visualized via negative contrast.
All tissues have unique relaxation times, which enables the production of different images. These images are based on the differences in the proton density and physicochemical properties. For instance, adipose tissues have shorter relaxation times compared to tissues with greater water content, which enables imaging of potential inflammation, tumors, and strokes.
MRI Technology Identifies NPs In Vivo
The blood-brain barrier (BBB) has been associated with the transmission of various neurologic therapeutics. These allow the passage of molecules of a molecular weight greater than 400 Da from the blood to the brain, which reduces the efficacy of multiple neurotherapeutic agents and biomarkers. Several strategies have been developed, such as direct surgical injection, active efflux transporter-targeted approach, intranasal delivery, and magnetic resonance-guided laser ablation techniques, to alleviate the issues associated with BBB during drug delivery.
Magnetic NPs are used as delivery vehicles for genes, drugs, and cell replacement therapies. These NPs are also used as contrast agents for MRI analysis, which helps visualize tumor angiogenesis in rats, bone marrow cells, neural stem cells, and other cells in vivo. It is important to investigate the biodistribution of magnetic nanoparticles after being introduced into a body.
Typically, the serum iron levels increase for several weeks after magnetic nanoparticle exposure and gradually decrease due to accumulation in the liver, brain, kidney, and heart or get degraded. Magnetic NPs are difficult to track via histological sections stained with hematoxylin, eosin, or iron dye.
A recent study investigated the use of MRI to visualize magnetic NPs in vivo. This study revealed that magnetic nanoparticles could be detected non-invasively even after 5 weeks of exposure. The key outcome of this study was the application of MRI to track nanoparticles in vivo in the eyes. The nanoparticle size is the primary determining factor in identifying its ocular presence. This study revealed the importance of nanoparticles size for its detection in vivo , which must be further explored in the future.
References and Further Reading
Reagen, S. and Zhao, J.X. (2022). Analysis of Nanomaterials on Biological and Environmental Systems and New Analytical Methods for Improved Detection. International Journal of Molecular Sciences. 23(11). p. 6331, https://doi.org/10.3390/ijms23116331.
Plaksin, M. et al. (2022) Magnetic resonance imaging analysis predicts nanoparticle concentration delivered to the brain parenchyma. Communications Biology. 5(1), p. 964. https://doi.org/10.1038/s42003-022-03881-0
Farinha, P. et al. (2021) A Comprehensive Updated Review on Magnetic Nanoparticles in Diagnostics. Nanomaterials (Basel). 11(12), p. 3432. https://doi.org/10.3390/nano11123432.
Angeloni, L. et al. (2018) Identification of nanoparticles and nanosystems in biological matrices with scanning probe microscopy. Nanomedicine and Nanobiotechnology. 10(6), p. e1521. https://doi.org/10.1002/wnan.1521
Estelrich, J. et al. (2015) Nanoparticles in magnetic resonance imaging: from simple to dual contrast agents. International Journal of Nanomedicine. 10, pp. 1727–1741. https://doi.org/10.2147%2FIJN.S76501
Raju, B.H. et al. (2012) Investigation of nanoparticles using magnetic resonance imaging after intravitreal injection. Clinical and Experimental Ophthalmology. 40(1), pp. 100–107. https://doi.org/10.1111/j.1442-9071.2011.02651.x
Disclaimer: The views expressed here are those of the author expressed in their private capacity and do not necessarily represent the views of AZoM.com Limited T/A AZoNetwork the owner and operator of this website. This disclaimer forms part of the Terms and conditions of use of this website.