As global energy demands continue to rise, developing improved energy storage solutions has become a pressing challenge. Nanomaterials have shown great promise for enhancing the performance of batteries, supercapacitors, and other electrochemical energy storage devices. However, several important practical factors must be considered before nanomaterials can be successfully implemented in commercial energy storage applications.
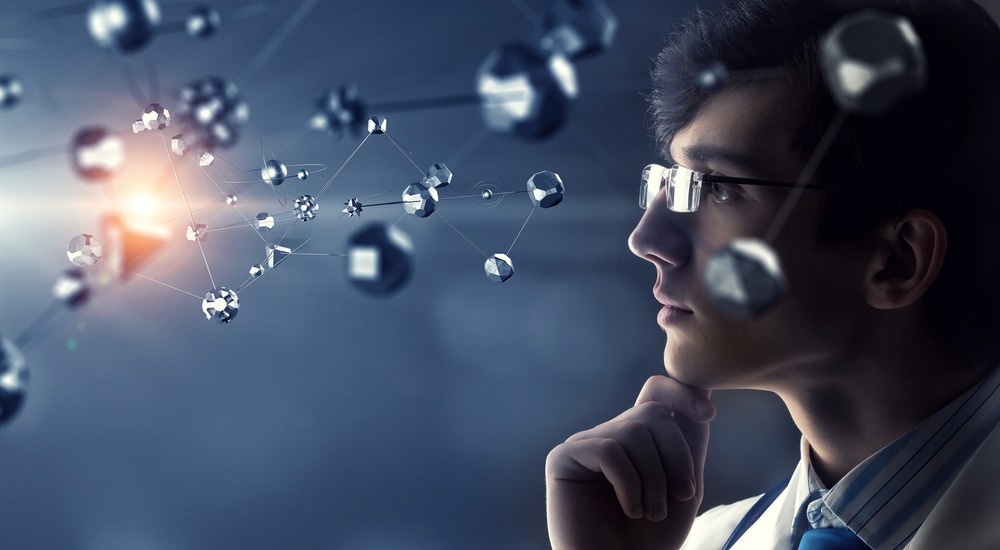
Image Credit: Sergey Nivens/Shutterstock.com
Nanomaterials are well-suited for energy storage devices due to their diverse properties, including high electrical conductivity, improved charge carrier mobility, compact size, and extensive surface area, which collectively enhance electron transport, storage efficiency, and charge/ion storage capacity.
Integrating nanomaterials, particularly in Li-ion batteries, has led to substantial advancements, expanding their application to technologies like flexible and wearable electronics. Additionally, nanomaterials come in various forms, and developers often combine different nanomaterials to achieve synergistic effects, enhancing device efficiency.
While nanotechnology-driven energy storage devices hold great promise for larger systems like electric vehicles, they are currently more prevalent in portable and handheld devices.
Practical Considerations for Integrating Nanomaterials into Energy Storage Devices
Nanotechnology is a double-edged sword; while it offers numerous benefits to energy storage devices, it can simultaneously introduce additional challenges.
Cost and Availability
One of the biggest challenges impeding the widespread adoption of nanomaterials is their high cost compared to conventional electrode materials. Materials like graphene, carbon nanotubes, and metal oxide nanowires are undoubtedly superior in properties. However, their complex multi-step synthesis procedures result in low yields and high costs.
For example, high-quality graphene made by chemical vapor deposition can cost over $1000 per gram. In comparison, graphite costs just a few cents. These high costs stem from the difficulty of scaling up nanomaterial synthesis processes.
Conventional lithium-ion batteries use micron-sized electrode powders that are relatively simple and cheap to manufacture in bulk. However, producing defect-free nanomaterials requires much more meticulous control over synthesis parameters. In addition, the reaction conditions, precursor materials, and processing steps need precise tuning to achieve high uniformity and purity. This results in low yield and throughput, driving up costs.
Scalability and Mass Production of Nanomaterial-Based Devices
Preliminary research on prototype devices in the lab has demonstrated remarkable improvements in performance by using nanomaterials. However, translating these to mass-produced commercial products requires solving engineering and manufacturing challenges.
Several aspects of the manufacturing process need re-engineering to work with nanomaterials. For example, ensuring uniform dispersion and stability of nanoparticles in solvents is critical but tricky. The mixing, coating, and drying steps may need modifications to prevent aggregation.
Compressing nanomaterial films into dense pellets requires investigation to avoid defects and cracks during assembly, especially if binders and processing are not optimized. In addition, quality control and characterization techniques must be tailored to analyze nanoscale morphologies and compositions effectively.
Therefore, conscious R&D efforts are required to design equipment, processes, and parameters specifically suited for nanomaterial-based energy storage device fabrication.
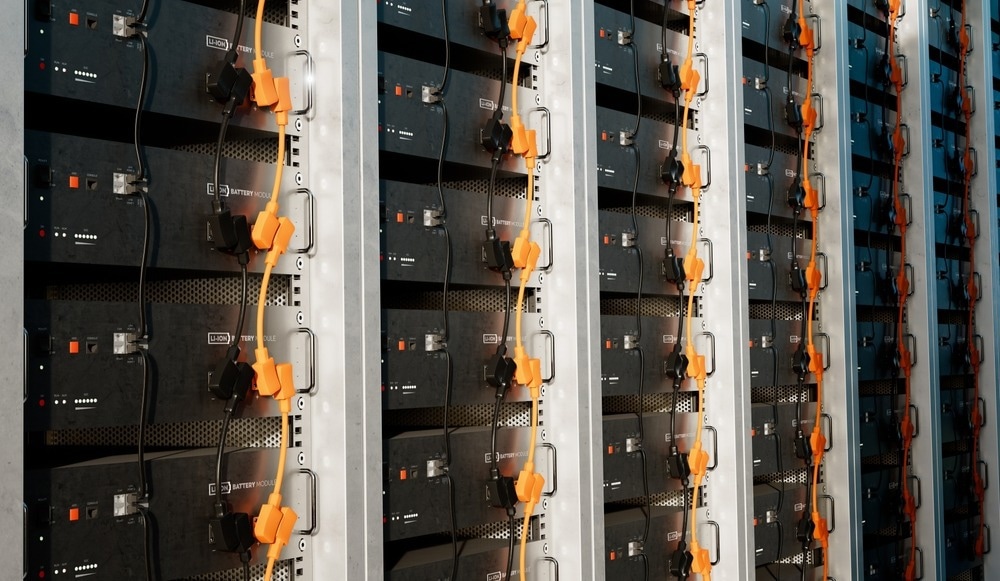
Image Credit: petrmalinak/Shutterstock.com
Stability and Durability
The high surface area of nanomaterials results in increased reactivity and side reactions, which can reduce device stability and storage lifetimes. For example, electrolyte decomposition reactions at electrode surfaces lead to capacity fading in batteries.
The increased electrode/electrolyte interfacial area of nanostructured electrodes causes solid electrolyte interphase (SEI) formation to consume more electrolytes and lithium. This results in low initial Coulombic efficiency and reduced battery capacity and energy density. A stable SEI is essential for electrode longevity, and controlling the specific SEI area is key to achieving high initial Coulombic efficiency.
Micrometer-scale secondary particles, featuring an outer electrolyte-blocking layer, reduce the specific SEI area, mitigating side reactions and enhancing initial Coulombic efficiency. A common example is the Si anode with a pomegranate-like design, which offers improved SEI control, fast electron transport, and reduced Si volume expansion, resulting in high performance and efficiency.
Surface passivation and coatings are employed to improve nanomaterial stability. Conformal metal oxide coatings on nanoparticles can serve as artificial solid-electrolyte interphases, reducing electrolyte side reactions while maintaining electronic and ionic conduction. Carbon coatings on nanowires can also improve cycle life by preventing material pulverization. However, many surface treatments require significant research to strike the right balance between stability and activity.
Rigorous evaluation of nanomaterials' mechanical and thermal resilience is crucial under repeated charge-discharge cycling to mimic real-world operation. If certain nanomaterials are less stable than conventional materials, robust encapsulation techniques may be necessary to enhance their safety and stability.
Widespread Adoption Differences Based on Economics
The high cost of nanomaterial-enabled energy storage can hinder adoption prospects in developing countries versus developed nations. Inevitable trade-offs may be necessary for wider global implementation. For instance, hybrid designs combining nanostructured and conventional electrodes could balance cost and performance. In addition, selectively incorporating nanomaterials only for certain components instead of entire batteries is another option.
Shared facilities for nanomaterial production may also make the technology more accessible to developing countries. Rather than each company investing in its fabrication setups, centralized production with distribution could be cost-effective.
Finally, continued R&D to improve nanomaterial synthesis processes for better yields and reduced costs is imperative for global adoption. If costs can be reduced by 10-100x, nanotechnology can greatly impact energy storage worldwide. This will require persistent efforts by the scientific community over the next decade. However, the promise nanomaterials have shown makes it a goal worth striving for.
Toxicity and Environmental Impact
Incorporating nanomaterials into batteries can exacerbate the already significant environmental challenges related to battery manufacturing and end-of-life recycling. The small size of nanomaterials allows them to enter cells and tissues more easily, potentially leading to DNA, protein, and membrane damage. For example, inhaling TiO2 particles with an elemental size ranging from 2 to 5 nm can lead to pneumonitis at a concentration of 8.8 mg per cubic meter.
Therefore, rigorous regulatory testing and approval are essential before the commercial use of batteries containing new nanomaterials to ensure their environmental impact is properly assessed and managed.
Concluding Remarks
Nanomaterials provide exciting opportunities to push the frontiers of energy storage technologies to meet growing performance demands. However, concerted interdisciplinary efforts across manufacturing, safety, integration, cost, and sustainability are vital to translating nanomaterials from lab concepts to widespread commercial impact.
References and Further Reading
Nagpure, S. C., & Bhushan, B. (2016). Nanomaterials for electrical energy storage devices. Encyclopedia of Nanotechnology, pp. 2473-2485. doi.org/10.1007/978-90-481-9751-4_58
Saleh, H. M., & Hassan, A. I. (2023). Synthesis and characterization of nanomaterials for application in cost-effective electrochemical devices. Sustainability, 15(14), p.10891. doi.org/10.3390/su151410891
Critchley, L. (2022). Nanotechnology's Impact on Energy Storage Devices. [Online]. Available at: https://www.mouser.com/blog/nanotechnology-impact-energy-storage-devices
Sun, Y., et al. (2016). Promises and challenges of nanomaterials for lithium-based rechargeable batteries. Nature Energy, 1(7), pp.1-12. doi.org/10.1038/nenergy.2016.71
Zhang, F. (2017). Grand challenges for nanoscience and nanotechnology in energy and health. Frontiers in chemistry, 5, p.80. doi.org/10.3389/fchem.2017.00080
Zhao, X., & Lehto, V. P. (2020). Challenges and prospects of nanosized silicon anodes in lithium-ion batteries. Nanotechnology, 32(4), p.042002. doi.org/10.1088/1361-6528/abb850
Disclaimer: The views expressed here are those of the author expressed in their private capacity and do not necessarily represent the views of AZoM.com Limited T/A AZoNetwork the owner and operator of this website. This disclaimer forms part of the Terms and conditions of use of this website.