Replicating in a controlled way the extreme conditions found in stars or in the center of nuclear explosions that can trigger nuclear fusion is one of the most challenging scientific endeavors in the past several decades. Despite the steady progress in the field, physicists are pursuing novel approaches that can achieve nuclear fusion using compact laser-driven fusion reactors.
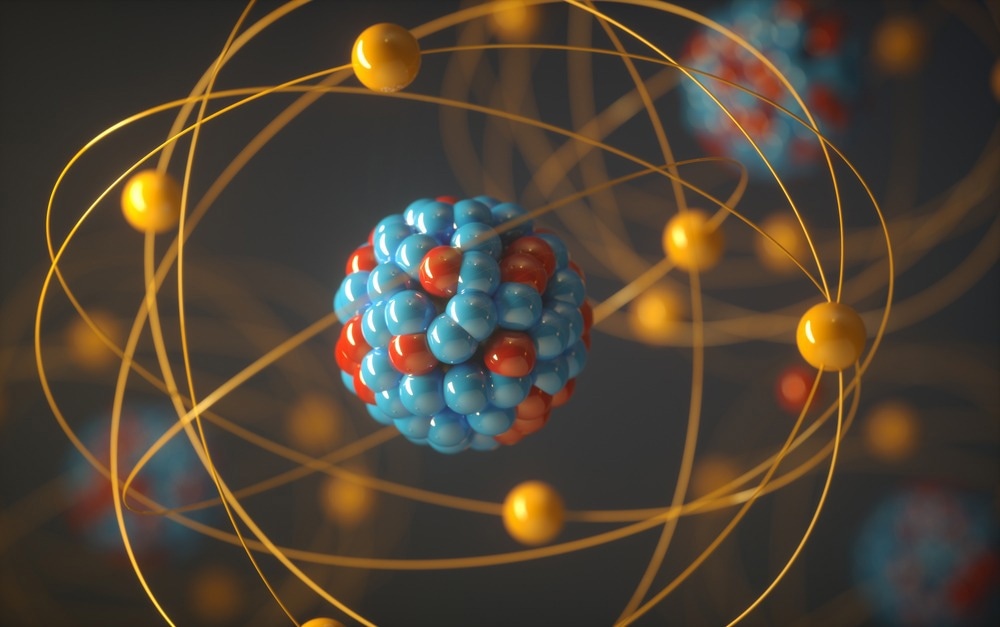
Image Credit: ktsdesign/Shutterstock.com
What is Nuclear Fusion and Why is it Important?
Nuclear fusion is a process where two nuclei of a light element, such as hydrogen or its isotopes, combine to form a single heavier nucleus (for example, helium) while releasing enormous amounts of energy. Fusion reactions power the stars where the matter is in a plasma state, a hot mix of positive ions and free electrons exhibiting unique properties very distinct from solids, liquids, and gases.
For the fusion reaction to occur, the nuclei must collide with sufficiently high energy corresponding to extremely high temperatures, between 10 and 100 million degrees Kelvin. The high temperature enables the charged particles to overcome the electrostatic repulsive forces. Once the separation between the nuclei becomes small enough (of the order of 10-15 m), the much stronger attractive nuclear force overcomes the electrostatic repulsion, causing the nuclei to fuse.
To facilitate the process, the nuclei must be confined within a small volume, or the plasma density needs to be sufficiently high to increase the collision probability. In the stars, the extreme pressure produced by their gravitational field creates the necessary conditions for nuclear fusion.
Since nuclear fusion fuel is abundant and relatively easily obtainable on Earth, the understanding of the nuclear fusion theory in the 1930s prompted physicists and engineers to research a way of harnessing it as a sustainable and affordable energy source. Nuclear fusion can generate four times more energy per kilogram of fuel than fission reactions, which is the energy source in nuclear power plants, and nearly four million times more energy than burning fossil fuels.
Inertial Confinement Approach for Nuclear Fusion
Currently, two main techniques for plasma confinement are employed in experimental fusion reactors worldwide. The magnetic confinement approach relies on generating strong magnetic fields to heat the plasma and ensure its stability over a sufficiently long period for the fusion reaction to proceed.
However, even using cryogenic superconducting magnets, the feasible magnetic field strength (a few Tesla) limits the achievable plasma density. As a result, most experimental fusion reactors have a plasma volume of a few hundred cubic meters, implying high construction and operational costs.
Inertial confinement operates in a pulsed mode, where small portions of fuel are compressed and heated by using powerful laser pulses so fast that a significant fraction of the atoms undergo fusion while the superhot plasma expands.
The energy released during the explosive process is limited by the mechanical, thermal, and radiation characteristics of the fusion chamber and is typically of the order of a few hundred megajoules.
The mass of a single inertial confinement fusion fuel pellet is just a few milligrams, while the compression and fusion reaction occurs on the timescale of several nanoseconds. Potentially, such compact fusion reactors utilizing small-size fuel pellets may be a viable route toward commercially viable nuclear fusion. However, the successful application of this approach is hindered by the hydrodynamic instabilities in the rapidly compressed plasma of the fuel pellet caused by the high-intensity laser beams.
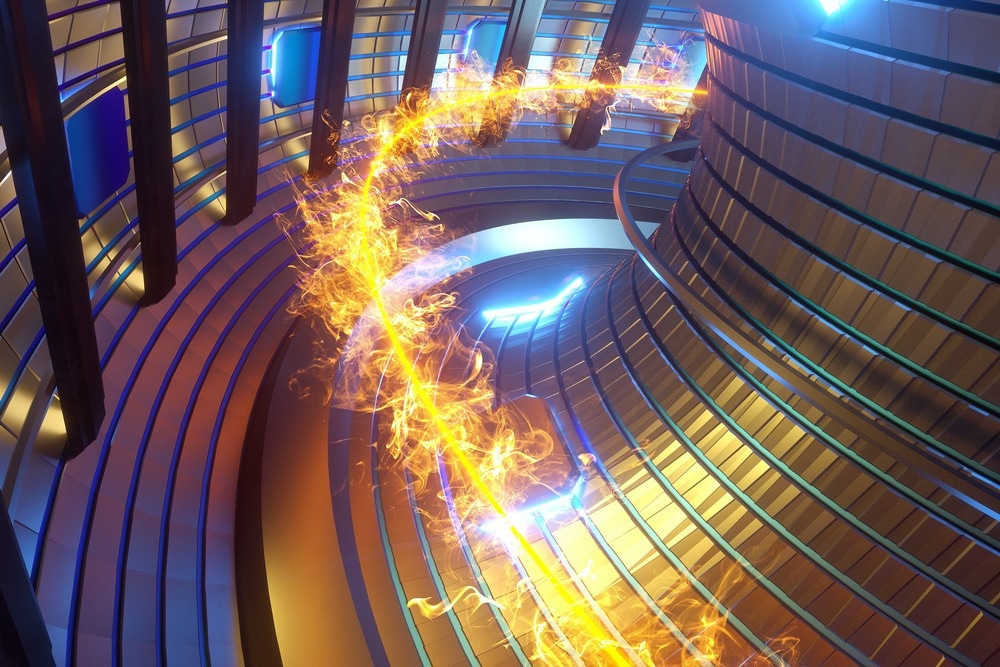
Image Credit: Marko Aliaksandr/Shutterstock.com
Nanomaterials for Laser-Driven Nuclear Fusion
A few years ago, a research group at Colorado State University led by Prof. Jorge Rocca demonstrated an important advance in inertially confined nuclear fusion using small-scale laser setups.
The researchers irradiated a nanostructured target containing thousands of highly ordered nanowires made of deuterated polyethylene, which is a material where deuterium atoms replace hydrogen, using femtosecond high-energy laser pulses.
The voids in the nanowire array enabled the laser radiation to rapidly penetrate the target where it is absorbed. The fast ionization of the target creates high-density superhot plasma where the deuterium nuclei are accelerated to energies in the megaelectronvolt range, thus triggering a deuterium fusion reaction. The reaction was accompanied by an extremely intense neutron flux of nearly 2 × 106 particles per joule of delivered laser power. These neutron bursts were more than two orders of magnitude stronger than those previously achieved using conventional targets of the same material.
Nanoplasmonics Ignites Nuclear Fusion
A recently formed nanoplasmonic laser inertial fusion experiment (NAPLIFE) research collaboration proposed another route toward achieving laser-driven nuclear fusion. The collaboration scientists, led by Dr. Tamas Biro at Wigner Research Centre for Physics in Hungary, proposed to use a plasmonic field enhancement effect to improve the laser energy absorption by the fuel target.
The idea is to disperse gold rod-shaped nanoparticles throughout the target material (deuterated polystyrene in this case). The incident laser radiation would excite plasmonic resonance (collective oscillation of the free electrons) in the nanoparticles.
The researchers estimate that, when the shape and structure of the nanorods are optimized, the plasmonic effect can increase the energy absorption of the composite target, a polymer with embedded nanorods, by nearly two orders of magnitude compared to a polymer-only target. The nanorods serve as resonant nanoantennas, which absorb and transfer the laser energy to the deuterated polymer of the target.
Each plasmonic nanoparticle acts as a hot spot that initiates the fusion reaction spreading throughout the target material. In this way, much shorter and less intense laser pulses can heat the target uniformly, ensuring that the entire volume of the target can reach ignition temperature.
The researchers continue to explore more exotic plasmonic nanostructures, like hollow spheres or torus-shaped nanoparticles, that can further enhance the energy absorption of the fuel target and bring us closer to achieving controlled nuclear fusion.
References and Further Reading
Papp, I., et al. (2022) Kinetic Model Evaluation of the Resilience of Plasmonic Nanoantennas for Laser-Induced Fusion. PRX Energy 1, p. 023001. Available at: https://doi.org/10.1103/PRXEnergy.1.023001
Jacquemot, S. (2017) Inertial confinement fusion for energy: overview of the ongoing experimental, theoretical and numerical studies. Nucl. Fusion 57. p. 102024. Available at: https://doi.org/10.1088/1741-4326/aa6d2d
Curtis, A., et al. (2018) Micro-scale fusion in dense relativistic nanowire array plasmas. Nat. Commun. 9, p. 1077. Available at: https://doi.org/10.1038/s41467-018-03445-z
Disclaimer: The views expressed here are those of the author expressed in their private capacity and do not necessarily represent the views of AZoM.com Limited T/A AZoNetwork the owner and operator of this website. This disclaimer forms part of the Terms and conditions of use of this website.